Nonequilibrium Ecology and Ecosystem Resiliency
The model for understanding ecosystems has long been based in equilibrium theory and linear succession. In recent decades, it has been recognized that climatic succession may be a concept well suited to some ecosystems that remain largely undisturbed, such as the nonlogged mesic forests of the Pacific Northwest. Most ecosystems throughout the world do not remain undisturbed, however, and therefore have perturbations (i.e., disturbances) that shift the ecosystem to alternate states. Nonequilibrium theory emerged as a way to think about ecosystems under the influence of disturbance and increased competition (Folke 2006). Nonequilibrium theory is now coupled with resiliency theory and resiliency thinking to recognize that ecosystems are not only under the influence of disturbance shifting; they are also subject to the influence of humans in and on ecosystems.
Section 1: Nonequilibrium Ecology
Nonequilibrium ecology asserts that ecosystems under disturbance pressure have a limited capacity for autogenic balancing and reach thresholds at which the system shifts to an altered state. An altered state likely includes a change in plant species composition and dominance and concomitant shifts in animal composition and ecosystem processes.
Equilibrium Systems vs. Nonequilibrium Systems
To better understand nonequilibrium ecology, let’s compare an equilibrium system to a nonequilibrium system. Briske et al. (2017) in Rangeland Systems: Processes, Management and Challenges provide us with a concise table comparison.
Type | Equilibrium systems | Nonequilibrium systems |
---|---|---|
Abiotic patterns | Relatively constant | Stochastic/variable |
Plant-herbivore interactions | Tight coupling | Weak coupling |
Biotic regulation | Abiotic drivers | |
Population Patterns | Density dependence | Density Independence |
Populations track carrying capacity | Dynamic carrying capacity limits population tracking | |
Community/ecosystem characteristics | Competitive structuring of communities | Competition not expressed |
Internal regulation | External drivers |
If we were to apply these characteristics to a real-life scenario such as an overgrazed and cheatgrass-invaded (disturbance) sagebrush perennial bunchgrass system, shifts in soil stability and hydrology (abiotic patterns) would be evident, limiting resources for the native community and opening up niches to which cheatgrass is well adapted. The vegetation community composition, through the external drivers of overgrazing and invasive species establishment and facilitated through feedback loops, crosses a threshold to shift from a native perennial bunchgrass community to a new state dominated by cheatgrass. This scenario is fairly well-known. What may not be as well-known, however, is that the process of transition from sagebrush perennial bunchgrass system to a cheatgrass-dominated system is nonequilibrium, but the altered state of cheatgrass dominance is a new state working toward equilibrium until another disturbance restarts the transition process.
So rather than thinking equilibrium versus nonequilibrium system, think about how stable systems are under the stress of disturbance shift; altered systems will reach a point of stability until another disturbance driver pushes them back to the previous system or to a new altered state. This is where resiliency theory comes in.
Section 2: Resiliency Theory
Resiliency is a system’s ability to autogenically either return to a high degree of predisturbance structure and function or move to a new stable, alternative state with similar function and structure. The driving objective of human land use and management is to maintain ecosystem integrity to deliver maximum ecosystem goods and services, which requires a high degree of continuity in system structure and function. This section will further explain resiliency theory, while the next section will provide a brief overview of resiliency thinking.
“Ball and Cup”
The diagram in figure 7.1, adapted from Briske et al. (2017), Rangeland Systems: Processes, Management and Challenges, illustrates the concept of resiliency.
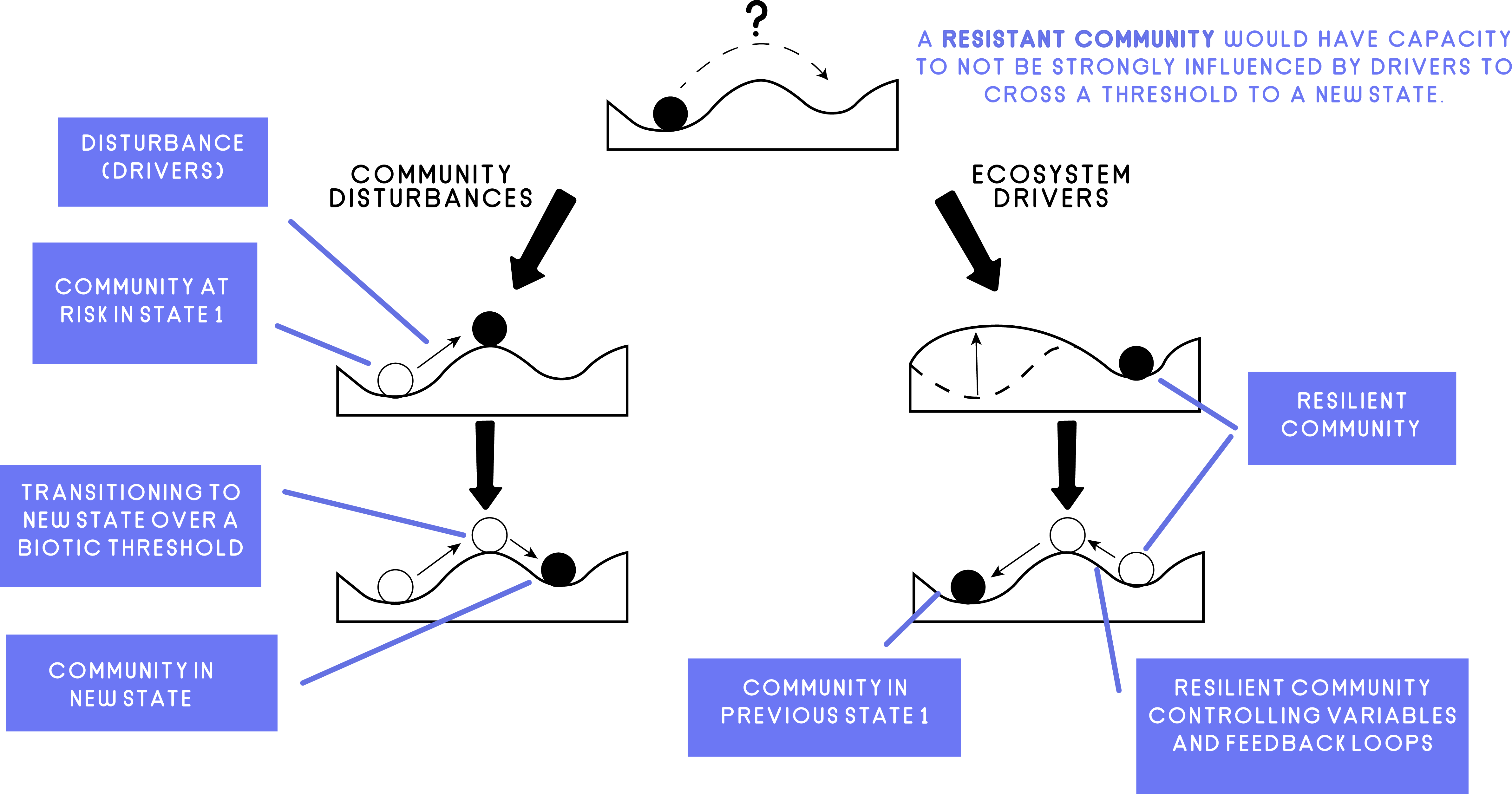
To solidify our understanding, let’s apply this model to a simplistic real-life scenario. A perennial bunchgrass-shrub community under heavy grazing and drought conditions (disturbance/drivers) crosses a biotic threshold to a new state with a shrub-dominated community. The perennial bunchgrass-shrub community had a high degree of ecological structure and function, as does the shrub-dominated community. After either a fire or the mowing of the shrubs, the system, because of its ecological integrity, reverts back to a perennial bunchgrass community.
In contrast, western juniper (Juniperus occidentalis) encroaches in a perennial bunchgrass community, which is also experiencing drought conditions (disturbance/drivers). As western juniper increases in density, the hydrology of the system changes, and the grass and forb community decreases, thus reducing soil quality and stability. This degraded system has a low level of resiliency and therefore crosses an abiotic threshold to a new state dominated by western juniper. After a fire or the felling of the western juniper, the ecological integrity of the system has been degraded to the point that it does not have the capacity to revert to any semblance of perennial bunchgrass community.
Key Elements of the “Ball-and-Cup” Model
To garner an understanding of how to make management decisions framed by the “Ball-and-Cup” model, one must have a grasp of elements: drivers, variables, and feedback loops.
Drivers are disturbances, both naturally occurring and anthropogenic in origin. Drivers disrupt the ecosystem’s structure and processes and are not integrated into the system’s organic feedback loops. Now fire may be an exception to this. Fire is a naturally occurring process that at low to moderate intensity and recurrence frequency can benefit a system. High-intensity fires or frequent fire occurrences, however, can be a disturbance driver that results in an altered nonresilient state.
Variables are ecosystem components, interactions, and processes. There are two types of variables: fast and slow. Fast variables reflect short-term cycles and immediate response to drivers. Examples include annual plant growth, seasonal soil hydrology, organism population fluctuations, and so on. Slow variables are the controlling variables in a system and are the greatest determinants of a system’s resiliency, as they influence fast variables and feedback loops. Slow variables are ecosystem attributes (see the outline in section 2.2).
Feedback loops are ecosystem processes that influence variable rates of change. There are two types of feedback loops—positive (amplifying and accelerating effect) and negative (stabilizing effect). Ecosystems with a high degree of resiliency are dominated by negative feedback loops, while ecosystems with a low degree of resiliency are dominated by positive feedback loops.
Attributes of and Influences on Ecosystem Resiliency
What are the attributes and influences that make an ecosystem resilient and that foster ecological integrity of structure and function? There are three categories of attributes and functions that indicate degree of resiliency: biotic integrity, soil stability, and hydrologic function.
Biotic Integrity
Biotic integrity is reflected by the degree of diversity in the system in terms of species composition, structure, function, and adaptations; genetic diversity, habitat diversity, and so on. Functional diversity (redundancy of species or groups of species functions in an ecosystem) is particularly important for resiliency, as it increases the system’s capacity to maintain balance and carry out ecosystem functions.
Soil Stability
Soil stability is reflected in maintained integrity of soil aggregate structure, bulk density, and belowground biomass and biotic functions. Soil type also influences ecosystem resiliency. The greater degree of soil development, and concomitant soil organic matter and available minerals, the greater the resiliency capacity.
Hydrologic Function
Hydrologic function is reflected in the system’s ability to capture, store, and safely release water. This ability is highly dependent on biotic integrity and soil stability.
Additional influences include climate and disturbance history.
Climate
Climate greatly influences a system’s ability to leverage its biotic integrity, soil stability, and hydrologic function. Hot, dry years can reduce plant production, which can reduce a system’s carrying capacity. Reduced plant production can reduce canopy cover and water interceptions and infiltration during precipitation events. Plant and soil evapotranspiration can increase, leaving soils with a fragile crust susceptible to erosion and reduced water infiltration. Reduced belowground plant rooting and microbial functions reduce nutrient cycling and increase bulk density.
Disturbance History, Frequency, Intensity, and Type
Disturbance history, frequency, intensity, and type (covered in chapter 6) also influence a system’s resiliency. An accumulation effect can build if the disturbance regime is frequent or if there is synergy among disturbance events.
Section 3: Resiliency Thinking and Social-Ecological Systems (SES)
Resiliency thinking is the integration of human understanding of nonequilibrium ecology, resiliency theory, and desire to maximize ecosystem goods and service delivery. It is a framework for humans in nature and a way of thinking that can help guide management, either by guiding change or by bolstering a system’s ability to recover post-disturbance. It is comprised of two approaches: (1) adaptive management and (2) social learning. These are elements in the overarching concept of social resilience.
Adaptive Management
Adaptive management is learning through doing. It is an approach that allows action to be taken in the face of uncertainty and to be adjusted based on progress, impacts, or outcome trajectories. Adaptive management provides flexibility to expand and contract the scope of management. This is of particular value, as interconnections previously not considered are illuminated and social and economic factors can be progressively included.
Social Learning
Social learning allows multiple stakeholders to contribute varying perspectives so that management decisions represent the ecological, social, and economic complexity of an ecosystem. The spirit of this approach aims not only to give all stakeholders a voice but also to learn from one another’s perspectives and interpretations to achieve the greatest degree of resiliency possible.
Social Resilience
Social resilience is a concept that emphasizes the integration of the social and economic into the focus on ecological resiliency. Social resilience is supported by the idea that socioeconomic systems that are resilient support communities or populations of people that have the capacity to thrive will in turn reduce anthropogenic pressures on natural resources and systems. It acknowledges that ecological, social, and economic factors are intricately tied together and of equal importance.
The ubiquity of human impacts and our dependence on the delivery of ecosystem requires resiliency thinking and a social-ecological framework. To achieve this, managers and decision makers representing multiple perspectives must work collaboratively to understand as completely as possible the autecology and synecology of ecosystems; the disturbance dynamics and resilient capacity of ecosystems; and the social-economic-economic connections of ecosystems.