5 Mitochondria and Chloroplasts
Structure-Function Relationships
Structure-Function Relationships
Introduction
The mitochondrion (plural mitochondria) and the chloroplast are fascinating organelles for a whole variety of reasons. Not only are they our primary energy producers, working together to capture light energy and then transforming it multiple times into various forms of chemical energy that we can use, but they have an origin story that is truly unique from the rest of the eukaryotic cell. On a global scale, mitochondria and chloroplasts are major contributors to the carbon cycle, in which carbon is taken up into the biosphere (through photosynthesis), cycled through multiple organisms as they interact (predation, herbivory, etc.), and then released back into the atmosphere (by cellular respiration) to start the cycle again.
The discussion of mitochondria and chloroplasts can get extremely complex quickly, as their primary function is to transform energy. Thus, much of the focus in introductory textbooks is on the biochemical reactions that take place during the process of photosynthesis and/or cellular respiration. In this textbook, we have chosen to take a different approach. Since this is a cell biology textbook, our focus will be on the structure/function relationships within these organelles rather than the biochemical reactions taking place. We will explore where these reactions occur and how the structural features of these two organelles support their function as energy producers. There are many other good open educational resources that explore the biochemistry of photosynthesis and cellular respiration. Suggestions include the following:
- Cellular Respiration Resources:
- Overview of cellular respiration (Amoeba Sisters): https://youtu.be/eJ9Zjc-jdys
- Molecular model of the mitochondrial electron transport chain and ATP synthase: https://youtu.be/LQmTKxI4Wn4
- Open Textbook Biology 2e chapter on cellular respiration: https://openstax.org/books/biology-2e/pages/7-introduction
- Photosynthesis Resources:
- Overview of photosynthesis (Amoeba Sisters): https://youtu.be/CMiPYHNNg28
- Molecular model of photosynthesis: https://youtu.be/jlO8NiPbgrk
- Open Textbook Biology 2e chapter on photosynthesis: https://openstax.org/books/biology-2e/pages/8-introduction
- Additional Open Biochemistry Textbook Resource on Energy Transformation: https://bio.libretexts.org/Bookshelves/Biochemistry/Book%3A_Biochemistry_Free_For_All_(Ahern_Rajagopal_and_Tan)/05%3A_Energy
If information on the biochemistry of these organelles is part of what you need to know, we encourage you to explore these and other options you may be aware of to supplement the information in this chapter.
Topic 5.1: Evolutionary Origins and Protein Targeting
Learning Goals
- Explain the evidence for the endosymbiont theory, which states that mitochondria and chloroplasts were once free-living bacteria.
- Compare and contrast the genomes of mitochondria and chloroplasts.
- Explain how proteins that are encoded by the nuclear DNA enter the mitochondria or chloroplasts.
- Compare and contrast protein targeting to mitochondria and chloroplasts with targeting to the nucleus and ER.
Mitochondria and Chloroplasts Evolved from Free-Living Bacteria
There has been a lot of debate over the years about the evolutionary origins of both mitochondria and chloroplasts, not to mention the origin of all of the other internal structures of eukaryotes (Figure 05-01). The fossil record tells us that prokaryotes, such as bacteria, evolved first, which means that somehow a prokaryote had to develop internal structures like the endomembrane system, nucleus, mitochondrion (and sometimes chloroplasts), and many other organelles that have not been observed in modern-day bacteria.
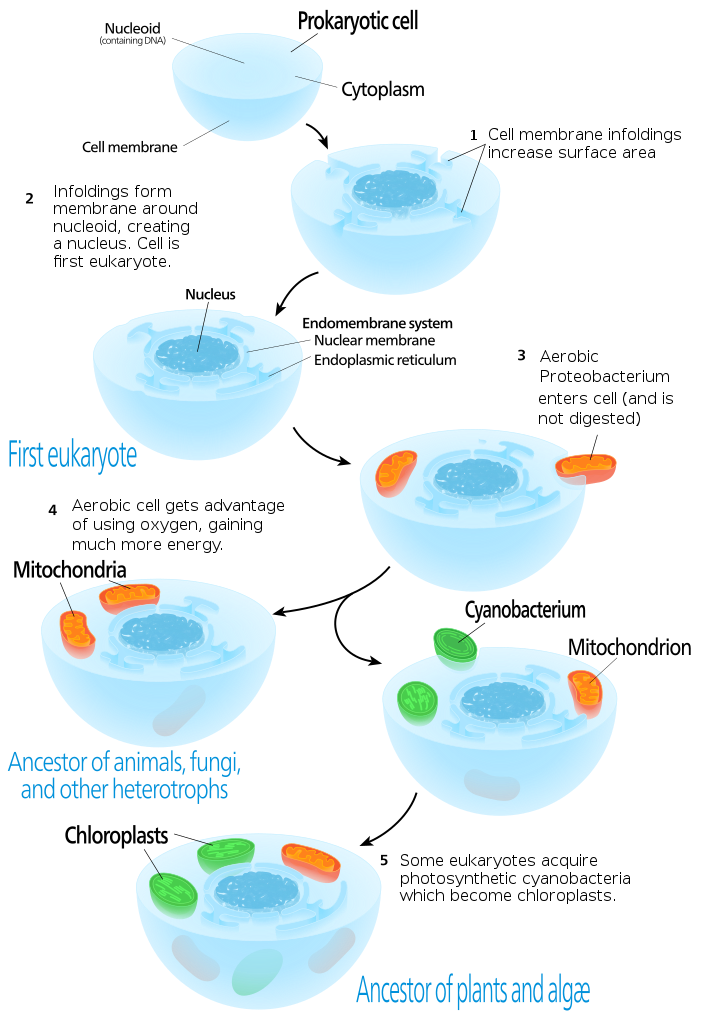
These days, the most widely accepted theory for the origin of the mitochondria and chloroplasts is a theory known as the endosymbiont theory. This theory was first proposed as far back as the late 1800s. It was based primarily on the observation that, much like bacteria, mitochondria and chloroplasts are able to duplicate themselves by binary fission. The endosymbiont theory was not widely accepted until, in the mid-1960s, it was discovered that mitochondria and chloroplasts contained DNA and ribosomes. This meant that they were able to synthesize at least some of their own proteins. This discovery, in addition to several publications by American scientist Dr. Lynn Margulis, who championed the theory, resulted in the eventual acceptance of the idea.
That is not to say that acceptance was easy. Dr. Margulis’s work was rejected and ridiculed in the 1960s, ’70s and ’80s. However, she persisted with promoting this theory, and her work on this topic is now considered to be revolutionary.
The endosymbiont theory is based on both the structural and genetic similarities among mitochondria, chloroplasts, and bacteria. Video 05-01 provides a simple summary of the principle behind the endosymbiont theory.
A Summary of the Evidence for the Endosymbiont Theory
There are several compelling arguments for the idea that mitochondria and chloroplasts were once free-living bacteria. These are based on a combination of structural and genetic similarities to specific bacterial species that exist today. (See Figure 05-02 for a structural comparison of a chloroplast and its nearest bacterial ancestor.) It should also be noted that the mitochondria and chloroplasts are thought to have derived from different bacterial ancestors at different points in the evolutionary timeline.
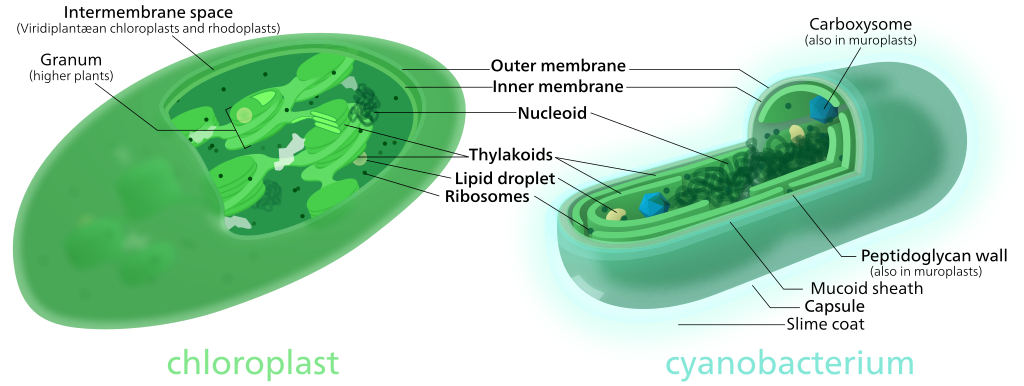
Below is a list of the most commonly mentioned similarities among mitochondria, chloroplasts, and their closest bacterial relatives, in no particular order:
- Just like bacteria, new mitochondria and chloroplasts can only be produced through binary fission.
- Binary fission is an asexual reproductive process where one cell splits into two cells.
- Because new chloroplasts and mitochondria can only be created through binary division, a cell’s mitochondria or chloroplasts cannot be re-created if they are all lost or destroyed. For example, in some algae, such as Euglena sp., chloroplasts are sometimes destroyed by exposure to specific chemicals or a prolonged absence of light. In such a case, the chloroplasts will not regenerate. However, as long as the cell has access to some kind of external carbohydrate source, they are able to survive and reproduce without them.
- There are several chemical components that are found in mitochondria and/or chloroplasts that are only found in bacteria and not in other parts of eukaryotes. For example,
- A membrane lipid called cardiolipin is exclusively found in the inner mitochondrial membrane and bacterial cell membranes but not other eukaryotic membranes.
- Proteins translated by mitochondria and chloroplasts use N-formylmethionine as the initiating amino acid, as do proteins created by bacteria. Eukaryotes use the unmodified form of methionine as the first amino acid.
- Transport proteins called porins are found in the outer membranes of mitochondria and chloroplasts and are also found in bacterial cell membranes.
- Mitochondria and chloroplasts most commonly carry circular DNA molecules that bear striking similarities to bacterial DNA (in both size and structure).
- Genome comparisons suggest a close relationship between mitochondria and bacterium known as Rickettsia sp.
- Chloroplast genome evolution appears to be slightly more complex (especially in the algae); however, there are many similarities between the genomes of higher plant chloroplasts and those of cyanobacteria.
Genomic Similarities of Mitochondria and Chloroplasts to Bacteria
In addition to the structural features described above, the genomes of mitochondria and chloroplasts also have many bacteria-like features as well as similarities in their protein-synthesizing systems. As mentioned, they have small circular DNA genomes, which are roughly comparable reduced versions of bacterial genomes. They synthesize some of the components of the electron transport chains used for oxidative phosphorylation (in mitochondria) and the light-harvesting photosynthetic systems (in chloroplasts). They also have ribosomes that are more similar to bacterial ones than they are to the ones in the cytosol of their own cell. Table 05-01 summarizes some of this information.
Some Properties of Bacteria, Mitochondria, and Chloroplasts as Compared to Eukaryotic Systems | ||||
---|---|---|---|---|
Trait | Gram-negative bacteria | Mitochondria | Chloroplasts | Eukaryotes |
Ribosomal RNAs | 23S, 16S | 23S or smaller, 16S or smaller |
23S or smaller, 16S or smaller |
28S, 5.8S, 18S |
Ribosomes | 70S total 50S, 30S subunits |
70S or smaller | 70S or smaller | 80S total, 60S, 40S subunits |
Genome | Circular DNA, no centromere |
Circular DNA, no centromere |
Circular DNA, no centromere |
Linear DNA with telomeres and centromeres |
Number of genes | ~5000 | 5–35 genes* | ~120 | 15,000– 100,000 |
Translation inhibitors | Chloramphenicol, streptomycin |
Chloramphenicol, streptomycin |
Chloramphenicol, streptomycin |
Cycloheximide |
*varies among eukaryotic groups |
The Mitochondrial Genome
Mitochondrial genomes are usually very small. The human mitochondrial genome is one of the smallest known, at only 16.8 kbp and 13 protein coding genes (plus some tRNA and rRNA genes). Brewer’s yeast (Saccharomyces cerevisiae) have a somewhat larger mitochondrial genome, at about 86 kbp and 100 protein coding genes. The model flowering plant, Arabidopsis thaliana, has a 367 kbp mitochondrial genome, which is more than three times the size but still only includes 86 genes. By comparison, the genome of modern-day Rickettsia prowazekii, a bacteria that is considered to be the closest living relative of mitochondria, is around 1100 kbp and includes 834 genes. Despite the difference in the size between the genomes of mitochondria and R. prowazeckii, they are still considered to be extremely similar overall. As an example, they are both somewhat unique, compared to other bacteria, in that they lack genes for glycolysis. As a result, in eukaryotic cells, glycolysis happens only in the cytosol of the eukaryotic cell, and not in the mitochondria.
Some examples of the genes included on mitochondrial genomes are the following:
- specific components of the transcription/translation machinery (tRNAs, ribosomal proteins, and rRNA)
- specific components of the electron transport chain
- specific components of the ATP synthase
In general, the proteins encoded tend to be the hydrophobic subunits of membrane proteins. The proteins are translated on mitochondrial ribosomes and remain in the mitochondrion. Other mitochondrial proteins, no longer synthesized within the mitochondrion, will need to be transcribed in the nucleus, translated in the cytosol of the eukaryote, and then imported into the mitochondrion prior to function. However, that is a topic we will tackle a little later in this chapter.
Of course, there is some diversity among Eukaryotes as to what exactly is contained within their respective mitochondrial genomes. Organisms within the same kingdom tend to be more similar to each other overall. Most mitochondrial genomes are circular, especially within the animals, but there are some that are linear.
The Chloroplast Genome
Chloroplast DNA also forms a circular genome and encodes for a variety of important chloroplast genes, including the following:
- specific components of the transcription/translation machinery (tRNAs, ribosomal proteins, and rRNA)
- specific components of the ATP synthase
- the large subunit of RuBisCO, the key enzyme involved in carbon fixation
- roughly 20 components of the chloroplast electron transport chain, used for photosynthesis
The size of the chloroplast genome is typically in the range of 120 to 160 kb. There seems to be much less variability in the size of the chloroplast genome from species to species compared to the genomes of the mitochondria. This point is interesting, as the origins of the chloroplast appear to be much more complex. Considering that all cells that have a chloroplast also have mitochondria, it is clear that chloroplast endosymbiosis came after the events that resulted in the development of the mitochondrion. However, it is also clear that chloroplasts were acquired by additional means after the initial primary endosymbiosis event. Secondary endosymbiosis is when a cell engulfs a chloroplast-containing cell, such as a red or green algae, which then will develop into that organism’s chloroplast. Secondary endosymbiosis results in some unique features in the chloroplast, which can be identified. For example, a “primary chloroplast” will have two membranes that surround it; a “secondary chloroplast” is expected to have three or more membranes. It may also have other “leftover” structures in between its membranes that may represent parts of the engulfed cell. Secondary endosymbiosis is virtually unknown in land plants but is quite common in protists, and red and green algae.
What’s Missing from Endosymbiont Organelle Genomes?
When we consider the size difference between the genomes of mitochondria and chloroplasts compared to their bacterial relatives, there is a question that arises and must be considered: What happened to the rest of the genes required for organellar function?
There are two possible answers based on the evidence we currently have:
- Some genes were probably lost over time, as their functions were no longer essential to survival in the intracellular environment. Alternately, these functions were essential but were already being carried out by the host cell, so the bacterial version was no longer essential. Genes coding for proteins involved in defense would fit into this category, for example.
- Other genes from mitochondrial or chloroplast genomes have, over the course of time, been transferred to the eukaryotic nucleus and incorporated into the nuclear genome.
The net result is that the majority of mitochondrial or chloroplast proteins are now encoded by nuclear genes. In the case of mitochondria, only a few highly hydrophobic protein subunits are encoded by mitochondrial genes and synthesized on ribosomes in the mitochondria. This means that almost all proteins in mitochondria or chloroplasts require the same kind of targeting system that we have seen for the nucleus and endomembrane system. The mitochondrial proteins encoded in the nuclear genome will be synthesized on free ribosomes in the cytosol and are then targeted to mitochondria or chloroplasts. We will end this topic by exploring protein import into these organelles.
Structural Features of Modern Mitochondria and Chloroplasts
While the current consensus, based on the evidence described above, is that mitochondria and chloroplasts have bacterial origins, it’s also quite clear that they have evolved a great deal since then. The structure of both organelles is reminiscent of their bacterial ancestors, but it is not identical. Figure 05-03, below, highlights the structural characteristics of each of these organelles.
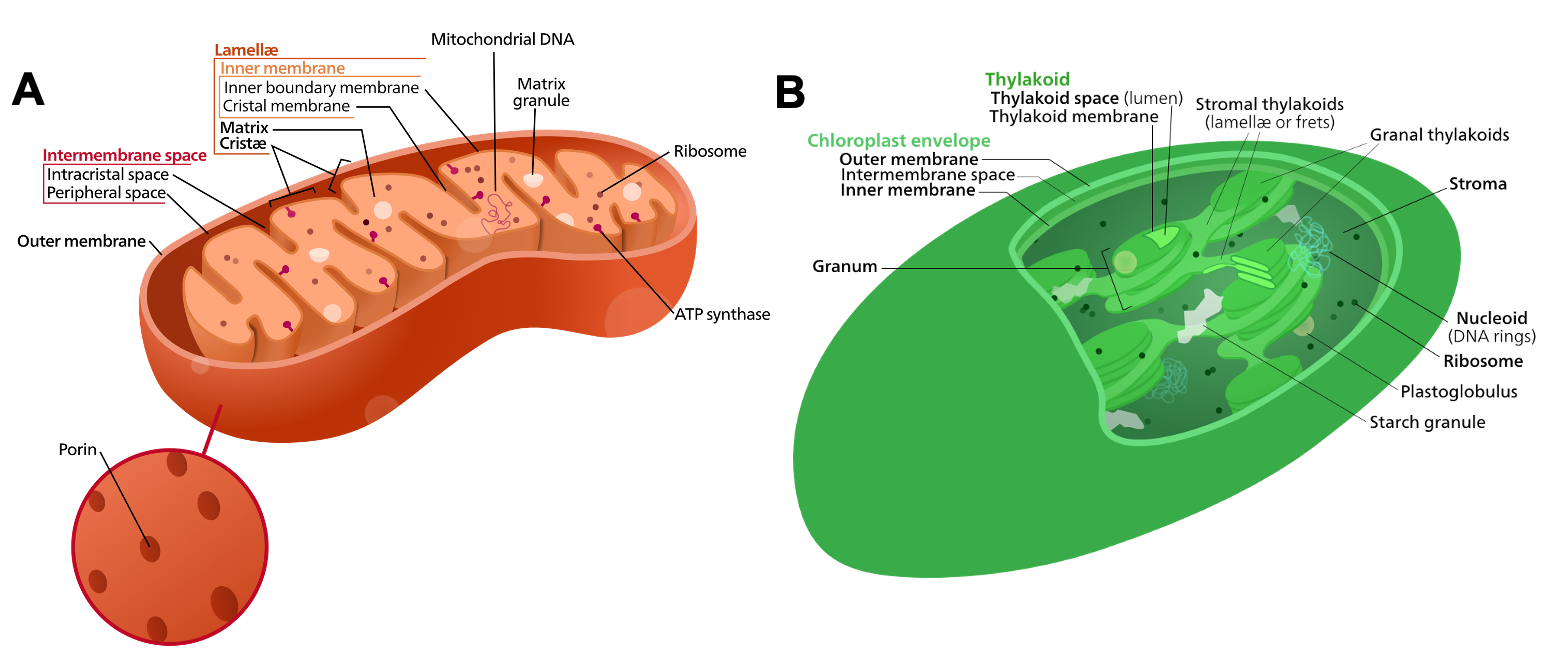
It’s worth taking a moment to study each of these images and to refer back to them as needed. In the rest of the chapter, we will be using much of this terminology to describe the location of the proteins and processes of each organelle, so it is important that you are familiar with them. Then in Topic 5.3, we will do a deeper dive into the structure of each of these organelles and how they function.
Protein Import into Mitochondria and Chloroplasts
The import of proteins into the mitochondria and chloroplasts follows the same general pattern that we’ve already seen in both the nucleus (Chapter 3) and the endomembrane system (Chapter 4). To remind you,
- The newly translated protein contains a targeting sequence, which identifies that it needs to be relocated.
- The targeting sequence is recognized by some kind of receptor that helps move the protein to the organelle in question.
- The protein enters the organelle, usually through some kind of translocation channel or pore.
Considering the structure of mitochondria and chloroplasts, targeting to these organelles can be quite complex. For example, a soluble protein that is destined for the matrix of the mitochondria will require one set of targeting sequences, whereas a protein that is destined to be embedded in the membrane of the cristae will require a different set of targeting sequences. In the chloroplast, there’s a third membrane, called the thylakoid membrane. Consequently, targeting to the chloroplast is even more complex than mitochondrial targeting, since there are more membranes, which results in more compartments. It would be impossible for us to cover all of this complexity in a textbook of this kind, so if this is a topic that you are interested in, here are some relatively recent review articles for you to explore: this one is on mitochondrial targeting (Wiedemann & Pfanner, 2017), and this one is about chloroplast targeting (Bölter & Soll, 2016).
There are only two import scenarios that we are going to consider in this section:
- Nucleus-encoded proteins that are destined for the mitochondrial matrix
- Nucleus-encoded proteins that are destined for the chloroplast stroma (with some bonus information about how proteins then go to the thylakoid lumen)
Targeting to the Mitochondrial Matrix
Our discussion begins with the targeting sequence used to identify that a protein is destined for the mitochondrial matrix. Like the other targeting sequences we have seen before, it is an amino acid sequence, which is included in the primary sequence of the protein. Key features of the targeting sequence include the following:
- It is always at the N-terminus of the protein so that it exits the ribosome first during translation.
- It has a very specific amino acid sequence (which we are not going to expect you to know).
- It shapes itself into an alpha helix after it is translated.
- The alpha helix acts exactly like a 3D key, which fits perfectly into a binding pocket on the import receptor protein.
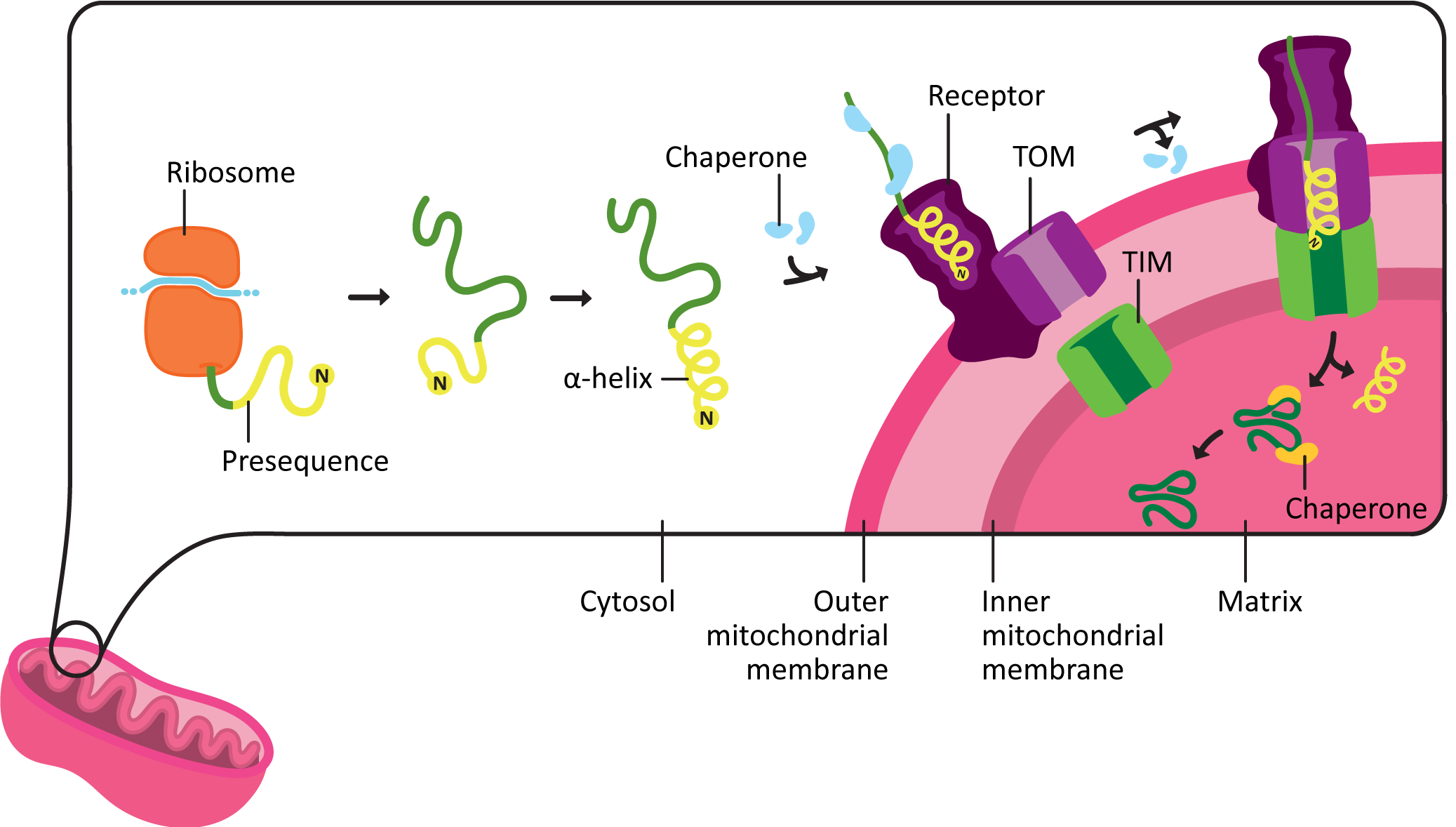
The import of mitochondrial matrix proteins happens roughly as follows:
- The protein containing the mitochondrial targeting sequence, known as a presequence, is synthesized entirely in the cytoplasm.
- The presequence is always located at the N-terminus of the protein and contains roughly 15–50 amino acids. This sequence is quite specific to make the correct shape once it is translated.
- As it emerges from the ribosome during translation, the presequence coils into an amphipathic alpha helix. This is an alpha helix that has one side that is polar, and the other is nonpolar. The shape and chemical properties of the presequence acts like a 3D key.
- The presequence binds to a receptor on the outer mitochondrial membrane. Chaperones help maintain the protein in an unfolded state until it can be transported into the mitochondria
- The protein is moved across the outer membrane, and then the inner membrane, through a pair of translocation channels known as TOM (transporter outer mitochondrial membrane) and TIM (transporter inner mitochondrial membrane).
- This process requires energy. A combination of ATP hydrolysis and harnessing the energy already stored in the proton gradient across the inner mitochondrial membrane is used to drive protein import. (We’ll talk about the proton gradient more in the next topic.)
- Chaperones assist with keeping the protein unfolded on the outside of the mitochondria, pulling the protein through the channel, and then folding the protein on the inside of the mitochondria.
- Once the protein is inside the matrix of the mitochondria, the presequence is removed by a proteolytic enzyme known as the mitochondrial processing peptidase, or MPP. After the presequence is removed, the protein is further processed and folded into its final mature shape.
Targeting to the Chloroplast Stroma
Chloroplast targeting is very similar to mitochondrial targeting. However, there are some unique features that are worth pointing out (Figure 05-04). For example,
- The chloroplast targeting sequence, known as the transit peptide, is also at the N-terminus of the protein, like the one for the mitochondrion. However, it’s not the same sequence. Plants must send different proteins to their mitochondria and chloroplasts, which means that the targeting sequences for these organelles also need to be different.
- The sequence of the transit peptide is highly specific, just like we saw in the mitochondria, but may also provide additional locational information to identify exactly where in the chloroplast the new protein is headed. For example, in Figure 05-04, we see that the transit peptide is immediately followed by a sequence that indicates the protein belongs in the thylakoid lumen.
- Like the mitochondrial presequence, it has a region that forms an alpha helix, which can act as a 3D key. Unlike the presequence, there is also a region that is unstructured whose function is not entirely clear but is likely involved in fine-tuning the targeting so that proteins can be sent to specific regions of the chloroplast.
- The transit peptide is recognized by a chloroplast import receptor on the surface of the organelle. Chloroplast proteins headed for the stroma are fed across two translocation channels called TOC (transporter outer chloroplast membrane) and TIC (transporter inner chloroplast membrane). This is, once again, an energy-requiring pathway, and ATP is consumed.
- Proteins destined for the thylakoid lumen will end up in the stroma at first (Figure 05-04). However, a second sequence is revealed at the N-terminus when the initial chloroplast targeting sequence is cleaved, which then directs the protein to the thylakoid lumen. We won’t be discussing this further, but it is very cool.
As you may have already guessed, the chloroplast transit peptide is also cleaved once the protein has entered the stroma. In this case, the protein responsible for the removal of the transit peptide is called the stromal processing peptidase, or SPP.
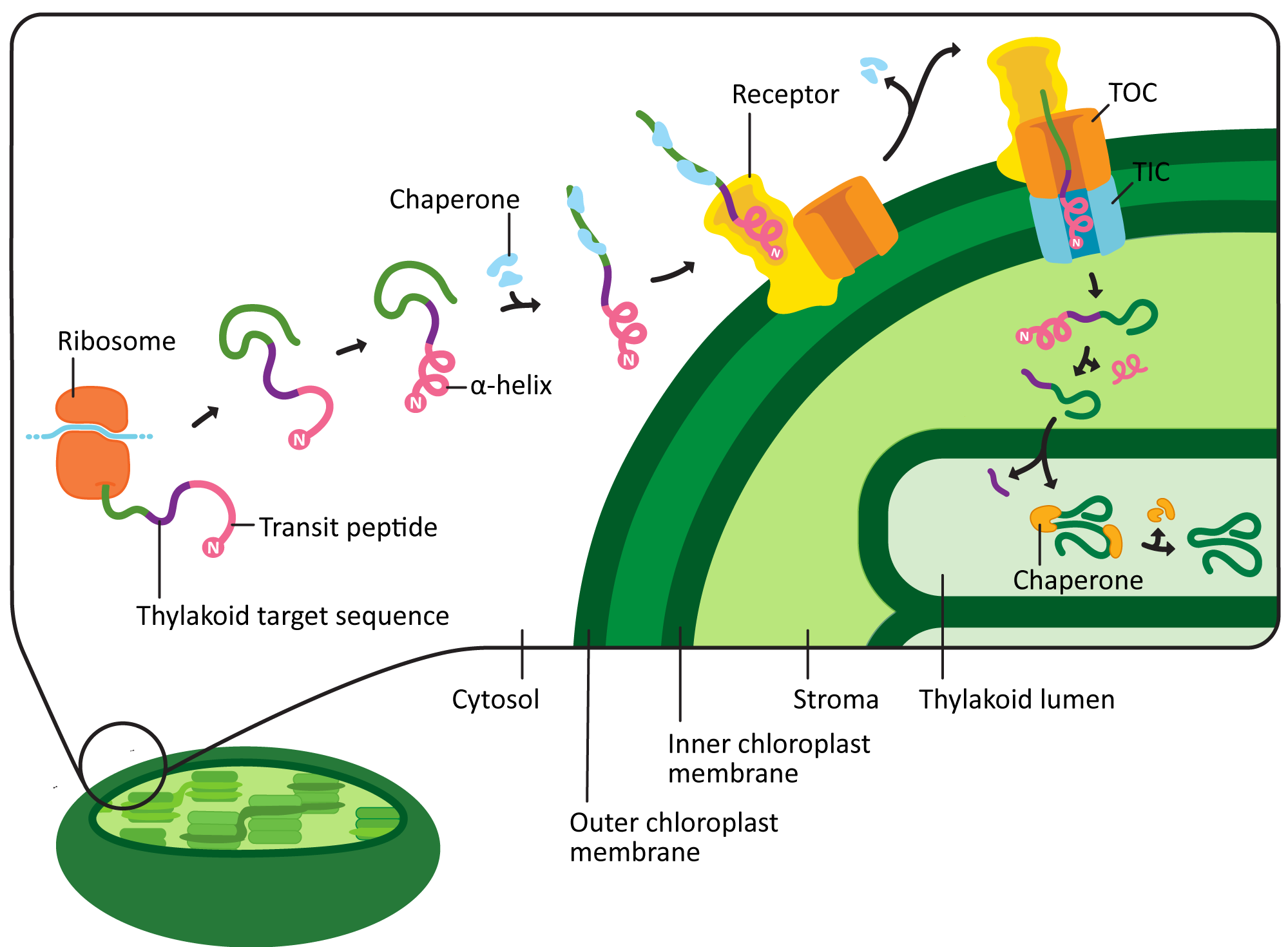
Topic 5.2: Function of Mitochondria and Chloroplasts
Learning Goals
- Compare and contrast chemiosmotic coupling of proton pumping and ATP formation in mitochondria and chloroplasts, and relate this to the ultimate functional goal of each organelle.
- Discuss the relationship between mitochondria and chloroplasts in all cells.
Introduction
As we have already explored in the previous topic, mitochondria and chloroplasts are thought to have evolved from bacterial ancestors and as such have some characteristics that are more aligned with bacteria than with eukaryotes. One of these similarities is how they produce ATP. However, the purpose of producing ATP in each of these organelles is completely different. This is a key point in understanding these organelles.
- The most important purpose of the mitochondria with respect to energy production is to produce ATP for the rest of the cell. Almost all known Eukaryotes have mitochondria, including photosynthetic plants and algae.
- On the other hand, the primary purpose of the chloroplast is to produce sugars via photosynthesis. While it also produces ATP within the organelle, all of the ATP produced in the chloroplast is used to build sugars (this is an energy-intensive process!). In fact, ATP made in the chloroplast is unable to leave the organelle, as it has no membrane transporters capable of ATP export.
The difference in function is extremely important to understanding these two organelles. In this section, we will first look at what’s similar in these organelles—namely, how they produce ATP. In the last topic, we will follow up by looking at the unique features of each organelle.
ATP Production in Mitochondria and Chloroplasts Happens via Chemiosmotic Coupling
As you likely know already, ATP is a vitally important biological molecule that is used to provide the chemical energy required to drive the chemical reactions catalyzed by our cells’ enzymes. You should also know that the mechanisms for generating ATP are ancient. For the most part, they predate the evolution of Eukaryotes, and if Eukaryotes had not found a way to harness the ATP-synthesizing abilities of bacteria and/or Archaea, they would not have survived.
There are three known ways of producing ATP, which fall into two major categories:
- Substrate-level phosphorylation. This is when a single enzyme is able to produce ATP by swapping an unstable phosphate from the enzyme’s substrate to ADP to produce ATP. Glycolysis produces all of its ATP via this method.
- Chemiosmotic coupling (Figure 05-06). In chemiosmotic coupling, a chemical gradient is generated by one set of membrane-bound protein. The gradient is used to help drive ATP production with a different set of membrane-bound proteins. In this way, these reactions are said to be coupled to produce ATP. This means that both are required for the system to function, and if either one is shut down for any reason, the other one will also stop functioning. In this kind of ATP generation, we see several membrane-bound protein complexes working together to produce ATP at a much higher rate than is possible via substrate-level phosphorylation. There are two main types that exist:
- Oxidative phosphorylation, done by the mitochondria. In this type, the oxidation of complex energy storage molecules (i.e., sugars, lipids, etc.) from food is used to provide energy to produce a proton gradient, which, in turn, is used to drive the synthesis of ATP.
- Photophosphorylation, done by the chloroplast. The premise is almost identical to oxidative phosphorylation, except that in the case of photophosphorylation, the source of energy that produces the proton gradient is the sun.
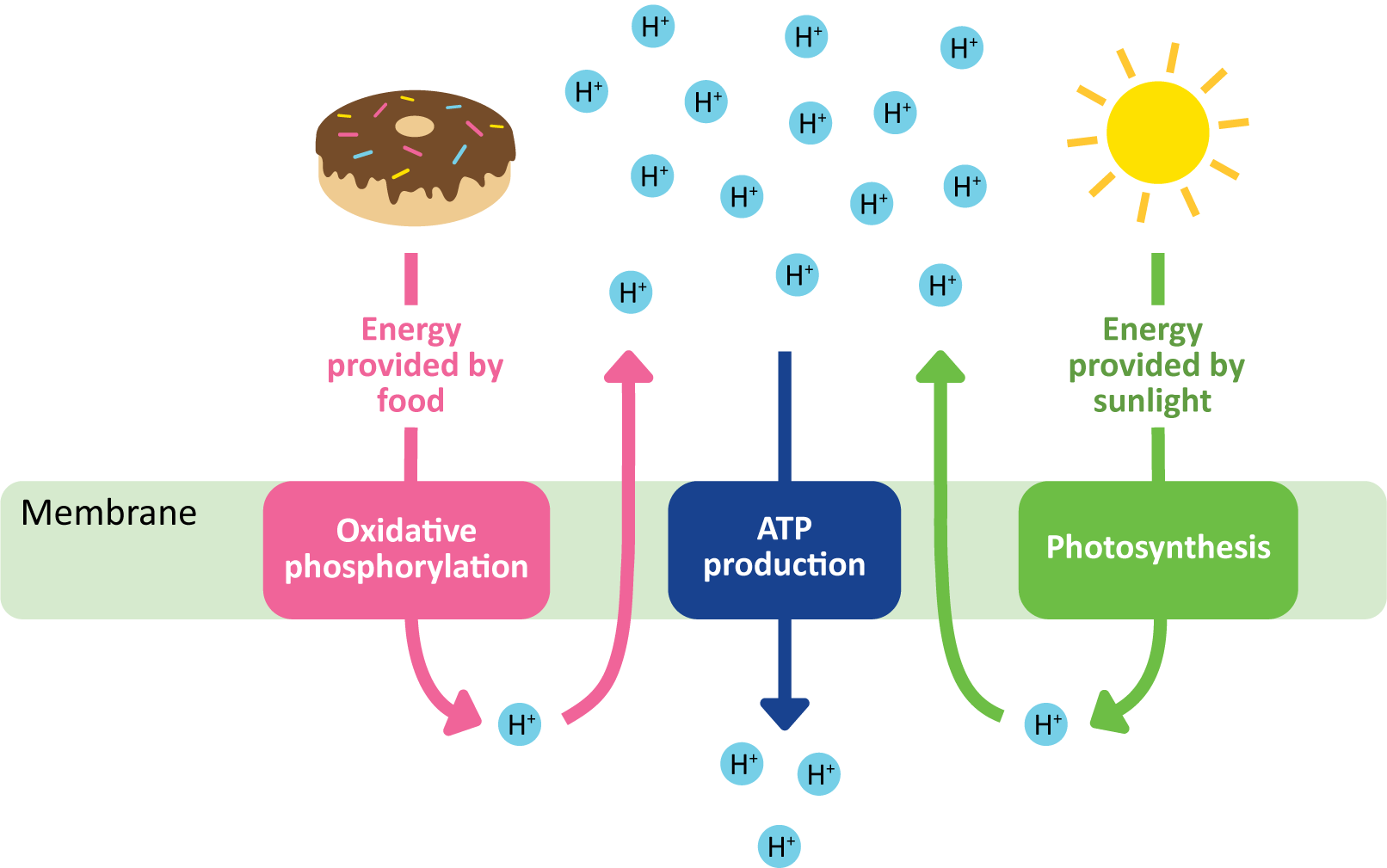
How Chemiosmotic Coupling Works
Substrate-level phosphorylation, which is used to make ATP in glycolysis, does not produce enough ATP to meet the energetic needs of most complex organisms (like Eukaryotes). We know this, in part, because there are no known multicellular organisms that rely entirely on glycolysis for their energetic needs. Chemiosmotic coupling is a process that is very ancient and widespread in the tree of life. Many of the proteins involved are highly conserved across kingdoms.
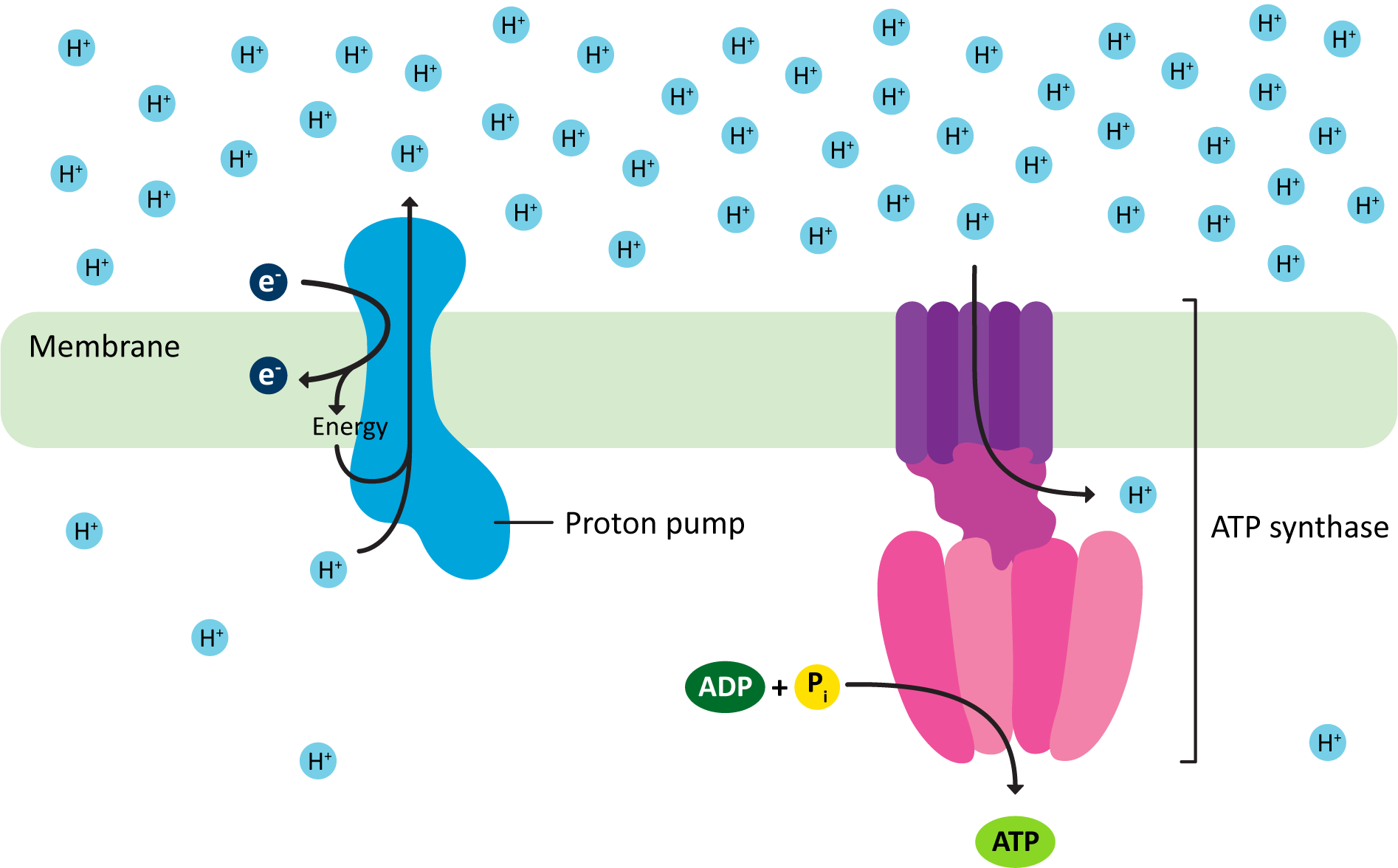
The key idea is very simple. It is a two-step process, which is visualized in Figure 05-07:
- A proton pump uses some kind of energy source to actively push protons (H+) across a membrane against their gradient. This produces an electrochemical gradient (meaning that there is both a chemical and charge difference across the membrane).
- The proton pumps of mitochondria and chloroplasts are each quite complex and consist of several large protein complexes that work together. Due to how they manage to build the proton gradient, we call them electron transport chains (ETCs). Again, while the premise here is similar, these ETCs are not the same.
- In mitochondria, the high-energy products of the citric acid cycle are fed into the ETC to drive the pumping of protons across the inner membrane of the mitochondria, whereas in the chloroplast, it is the energy of the sun, captured by chlorophyll, that helps drive the pumping of protons by the chloroplast ETC.
- The energy that was used to produce the electrochemical gradient is now stored within it as potential energy. We can calculate the energy stored in the gradient by measuring the pH on either side of the membrane and using that to convert to the amount of energy stored. (If you take biochemistry in the future, you should expect to learn how to do this calculation.)
- The energy contained in this electrochemical gradient is used to drive the phosphorylation of ADP to produce ATP. This occurs as protons move through a channel across the membrane that is part of the ATP synthase complex.
We call this particular usage of hydrogen ions, in which they are actively being transported against their gradient first so that they can then flow back down their gradient to do work, the proton-motive force. It is a crucial part of ATP production in both mitochondria and chloroplasts.
The ATP Synthase
The ATP synthase is key to the production of ATP in both the mitochondrion and the chloroplast. The ATP synthase is a very large protein complex that is embedded in either the inner mitochondrial membrane or the thylakoid membrane, depending on the organelle (Figure 05-08 and Video 05-02). This is the same enzyme used by aerobic bacteria to produce ATP. Versions of this protein are also found in other membranes, like the endomembrane system. However, outside of the mitochondria, they are not used for ATP synthesis but instead break down ATP to actively pump protons across the membrane to create the acidic environment inside of endosomes and lysosomes.
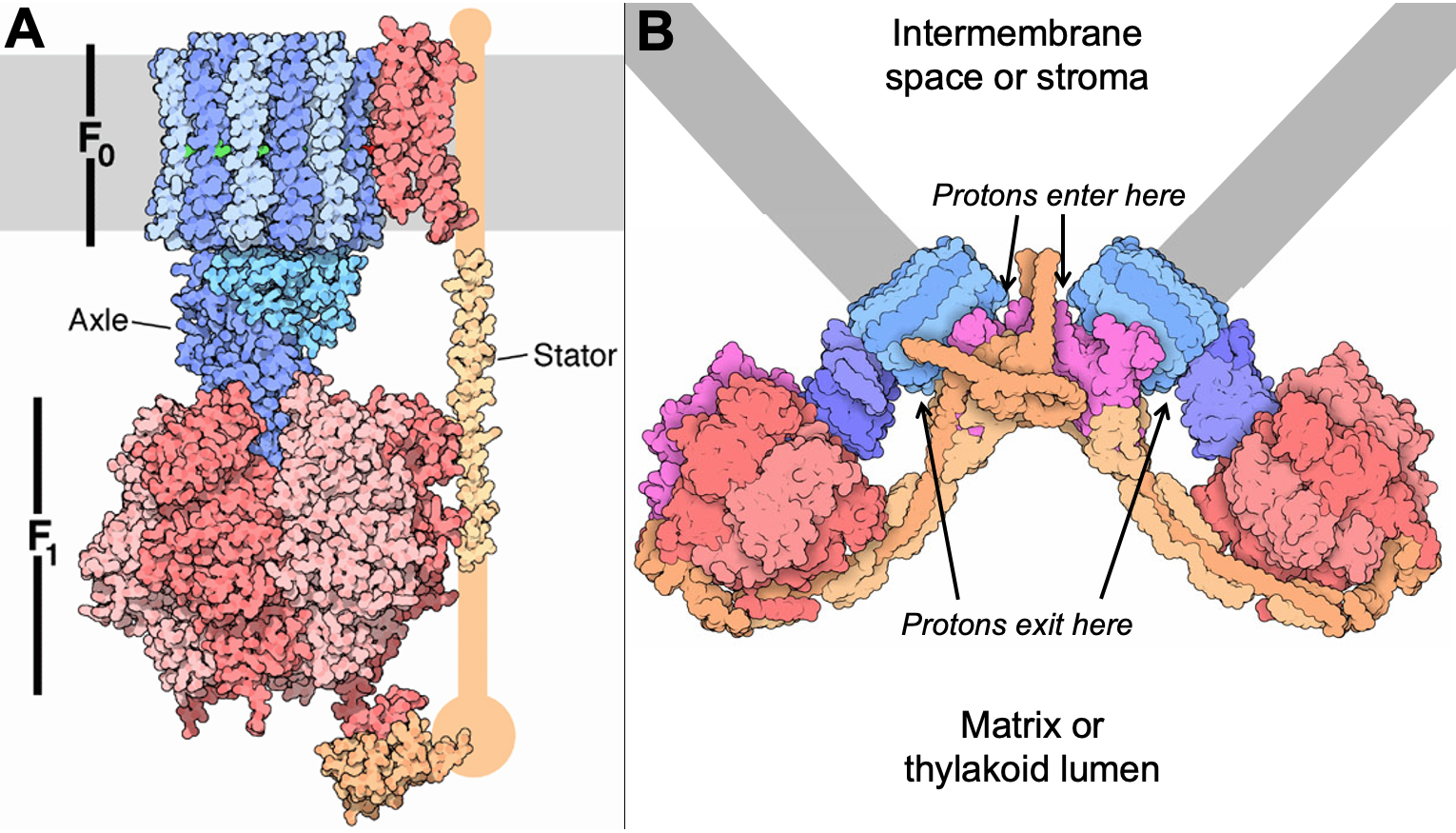
The ATP synthase is a truly unique molecular machine. There are very few biological examples of proteins that rotate in this way to create energy. And yet this is a highly effective and elegant way to generate the energy required to create ATP. The ATP synthase, and the electron transport chain that creates the proton gradient that powers it, is one of the most important factors in the success of Eukaryotes.
Chemiosmotic Coupling in Mitochondria and Chloroplasts Is Arranged Differently
It is important to note the differences in how the proton pumps and ATP synthase are arranged in each of the organelles. Part of the reason that they are this way is due to the bacteria that they evolved from. (Remember from the previous topic that the two organelles are thought to come from separate endosymbiotic events.) This is illustrated in Figure 05-09.
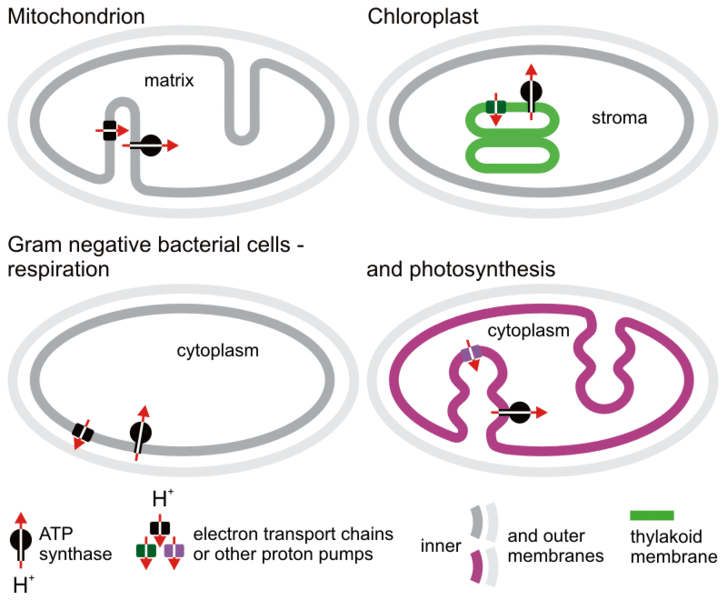
It’s important to point out a few things from Figure 05-09 that highlight key differences between mitochondria and chloroplasts. Most notably, mitochondria evolved from “simpler” bacteria than chloroplasts did. We see this, in part, in the arrangement of the internal membrane structures in each organelle. The chloroplast ancestor already had inward projections of its membranes, which eventually evolved into the thylakoid membrane. As such, chloroplasts have an additional set of internal membranes that are not present in mitochondria. This has resulted in differences in how the two organelles function:
- In mitochondria, the electrochemical gradient is built across the inner mitochondrial membrane (also known as the cristae). The difference in pH across that inner membrane where the ETC and ATP synthase reside is quite small (sometimes less than a pH point). This is because of the following:
- The gradient is produced by pumping the protons out of the organelle, so the matrix pH goes up and becomes more basic.
- The exterior membrane is porous (due to porins that allow free passage of water and solutes; see Figure 05-10 later in this chapter). So the protons will diffuse out of the intermembrane space into the cytosol over time by way of the porins. This means that even though the ETC is continuously pumping protons into the intermembrane space, there is no real accumulation on ions in the exterior compartment. So the pH of the intermembrane space remains roughly the same as the cytosolic pH.
- Despite this, the small change in pH creates a large enough difference in electrical potential that the ATP synthase can function.
- In chloroplasts, the thylakoid membrane is the site of the ETC and the ATP synthase.
- The protons are pumped into the thylakoid lumen. As such, the pH in that compartment is much lower compared to the stroma. Since the thylakoid lumen is an enclosed space (unlike the intermembrane space of the mitochondria, which is connected to the cytosol), a much more significant pH difference can be created in the chloroplast than the one produced by the mitochondria.
- Interestingly, the thylakoid membrane is highly permeable to Mg2+ and Cl– ions, which pass freely and are in abundance. This effectively destroys the electrical component of this gradient. So virtually all of the energy stored in this gradient comes from the difference in chemical concentration of protons across this membrane.
How Do the Mitochondrion and Chloroplast Work Together?
When undergraduate students are asked to learn about photosynthesis, a common question is often overheard: “OK, great. Photosynthesis gave us enough oxygen in our atmosphere, but what have plants done for us lately? I mean, I don’t even have a chloroplast, so why should I care about it?” Here we present some arguments for you to consider. Hopefully it will convince you that understanding plants and photosynthesis is more important than you may realize.
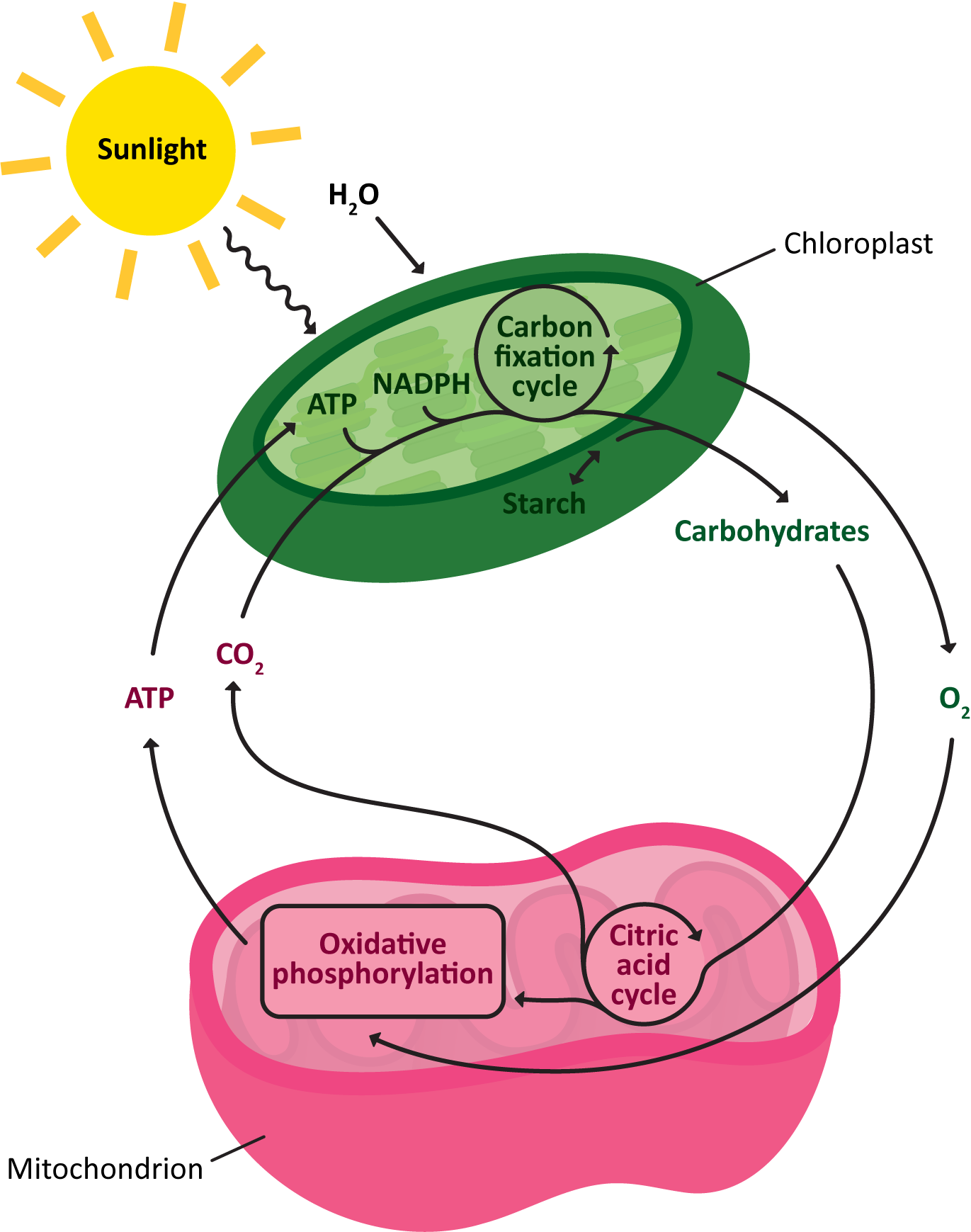
First and foremost, regardless of whether you have your own chloroplast or not, mitochondria and chloroplasts work together. Each one is required by the other in order to keep the energy (and carbon) flowing through the biosphere. There is a cycle that forms between the chloroplasts of plants and algae and the mitochondria of all living organisms (including those of plants and algae).
- The chloroplast uses carbon dioxide and water to produce oxygen and carbohydrates.
- The oxygen and carbohydrates are then used by the mitochondria, energy is released, carbon dioxide and water are produced, and the cycle continues.
On top of that, even though you don’t have a chloroplast, you absolutely do require its functions in order to survive. Plants are both autotrophs (meaning that they are capable of making everything they need to survive) and primary producers (meaning that they sit at the bottom of the food chain, and everything else is dependent on them). We, on the other hand, are heterotrophs (meaning that we cannot produce everything we need to survive) and are at the top of our food chain (meaning very few things prey on us for food). Without the primary producers, the entire food chain will fall apart. Without an apex predator like ourselves? Most likely a different apex predator will take our place, and no one will notice we’re gone. All of this is to say that we need plants and their ability to photosynthesize far more than they need us.
It is for this reason that cell biology textbooks such as this spend time teaching you about an organelle that you don’t even have…but wouldn’t it be cool if you did?
Topic 5.3: Structure-Function Relationships in Mitochondria and Chloroplasts
Learning Goals
- Identify mitochondria and chloroplasts in micrographs and differentiate them from other organelles and from each other.
- Explain how the structure of the mitochondrion contributes to its function as the primary source of ATP in the cell.
- Explain how the structure of the chloroplast contributes to its function as the primary source of sugars for plant cells and the primary site of carbon fixation on the planet.
- Compare and contrast the role of chloroplasts with the role of mitochondria.
- List additional functions of the mitochondrion and the chloroplast.
Topics 5.1 and 5.2 mostly focused on how the mitochondrion and chloroplast are similar. We explored their evolutionary origins, their protein targeting and import, and how they produce ATP. However, at this point in the chapter, it is clear that we also need to spend some time considering what sets each of these organelles apart from the other. We will look at the mitochondria first, followed by the chloroplast.
Mitochondrial Structure
It is unclear how the mitochondria became such an internet sensation, yet somehow it did. So it should come as no surprise to you that the mitochondria is the powerhouse of the cell. However, it’s important that you now step back and consider what that actually means from a cellular perspective. The mitochondrion is the primary producer of chemical energy in the cell, mostly in the form of ATP. A single organelle is known as a mitochondrion, whereas many of this organelle are known as mitochondria.
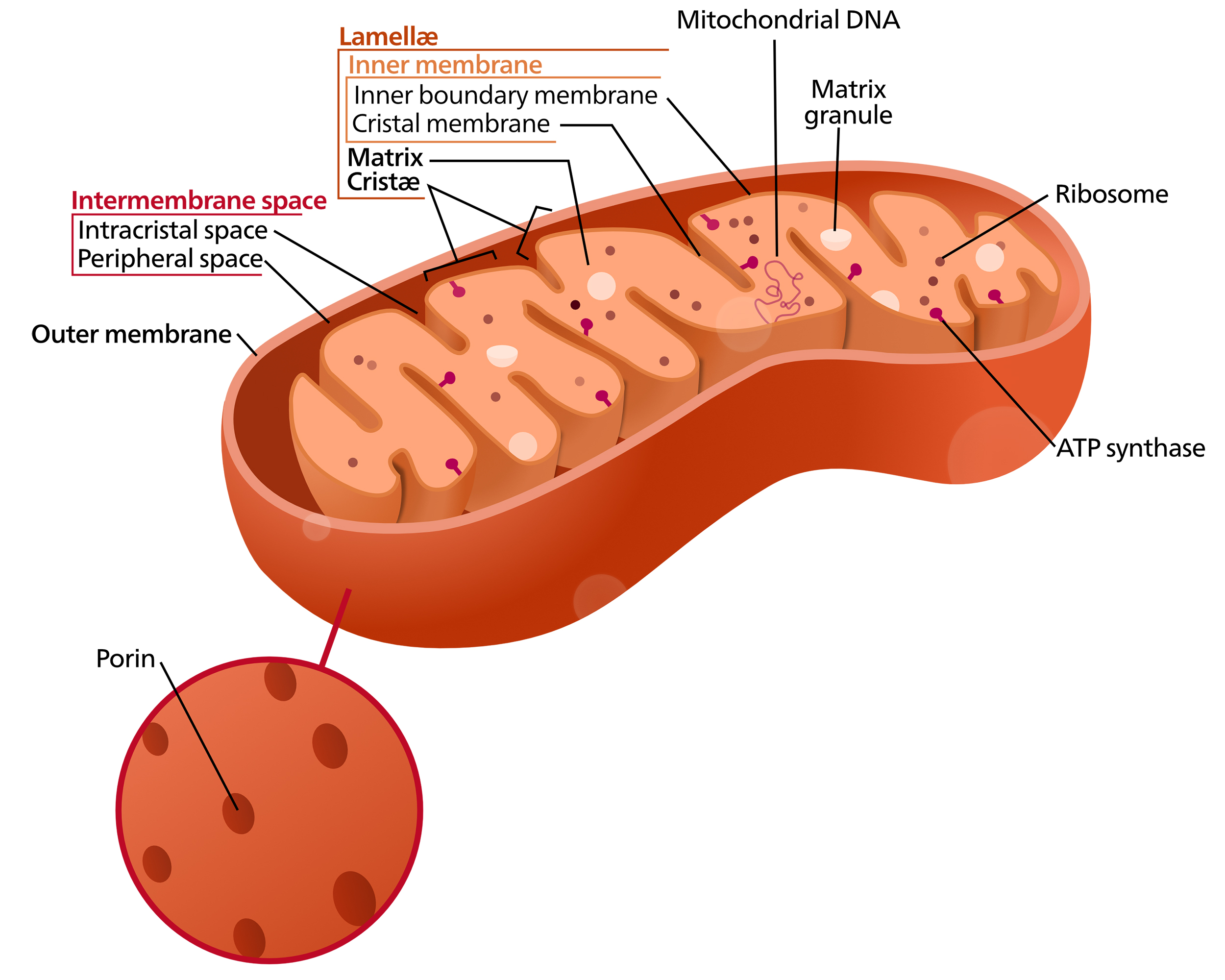
The mitochondrion has two exterior membranes that surround it (Figure 05-10). The “cytosol” inside the innermost membrane of the mitochondrion is called the matrix. The original bacterial plasma membrane corresponds to the inner mitochondrial membrane. The inner membrane of mitochondria has infoldings or invaginations called cristae (crista is the singular form). For the most part, cristae look more or less like you’ve seen in textbooks; however, that does not have to be the case. They can also be tubular or fingerlike projections into the matrix. As we discussed previously, the curvature of the crista membrane is the result of the dimerization of the ATP synthase, which we discussed earlier (Figure 05-07 and associated text).
The bacterial origins of the mitochondrion, along with transmission electron microscopy (TEM) images showing cross sections of the organelle, tend to give a false impression of the shape of a mitochondrion. Most folks think of the mitochondrion as a small and discrete structure with a very static kidney bean shape. This is not so. In fact, mitochondria can be quite versatile and dynamic, constantly fusing and dividing in a cell. Newer data from live-cell imaging show that mitochondria are extremely dynamic and constantly move, fuse, and split apart. They form long, interconnected networks that can extend throughout the cytoplasm, as you can see in Video 05-03 below. (FYI, the mitochondria are the little yellow inchworms. The nuclei are the large cyan balls on the left-hand side. It’s unclear what the red is from the information in this video.)
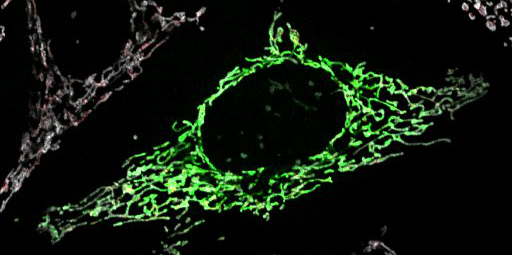
What you should be able to see in both Figure 05-12 and Video 05-03 (above) is that the mitochondria are very different in reality than the caricature we have of them in our heads (and in the internet memes). The rise of fluorescence microscopy (and now superresolution microscopy) has really changed our understanding of the structure of these organelles as well as how their dynamic nature might contribute to their function.
Mitochondrial Function
The mitochondrion has several functions, not the least of which is the production of ATP for the rest of the cell. We will not discuss the details of how that happens here, as we’ve already said everything that we want to say about this in Topic 5.2. Here, we are mostly interested in the structure-function relationships within the mitochondrion. In other words, we expect you to know where all of the steps of ATP generation take place within this organelle. Figure 05-12, below, summarizes the main steps and locates them nicely within the organelle.
Cellular Respiration and Oxidative Phosphorylation
While we are not discussing the process of cellular respiration in all of its biochemical detail, we will start this section by reminding you of the major steps, which are also identified in Figure 05-12:
- Glycolysis happens in the cytosol of the cell. It produces small amounts of ATP, but more importantly, it converts glucose into pyruvate, which is required for the next seps of the process.
- Pyruvate is imported into the mitochondrial matrix and is then converted into a molecule called Acetyl-CoA. In the process, some NADH is made.
- Acetyl-CoA is fed into the citric acid cycle (also known as the Krebs cycle), which creates more NADH and also a similar molecule called FADH2. These are both important electron carriers and are key to providing the energy required for the active transport of protons across the electron transport chain.
- The electron transport chain creates the proton gradient, which will then be used to synthesize many ATP, via the ATP synthase that was extensively covered in Topic 5.2.
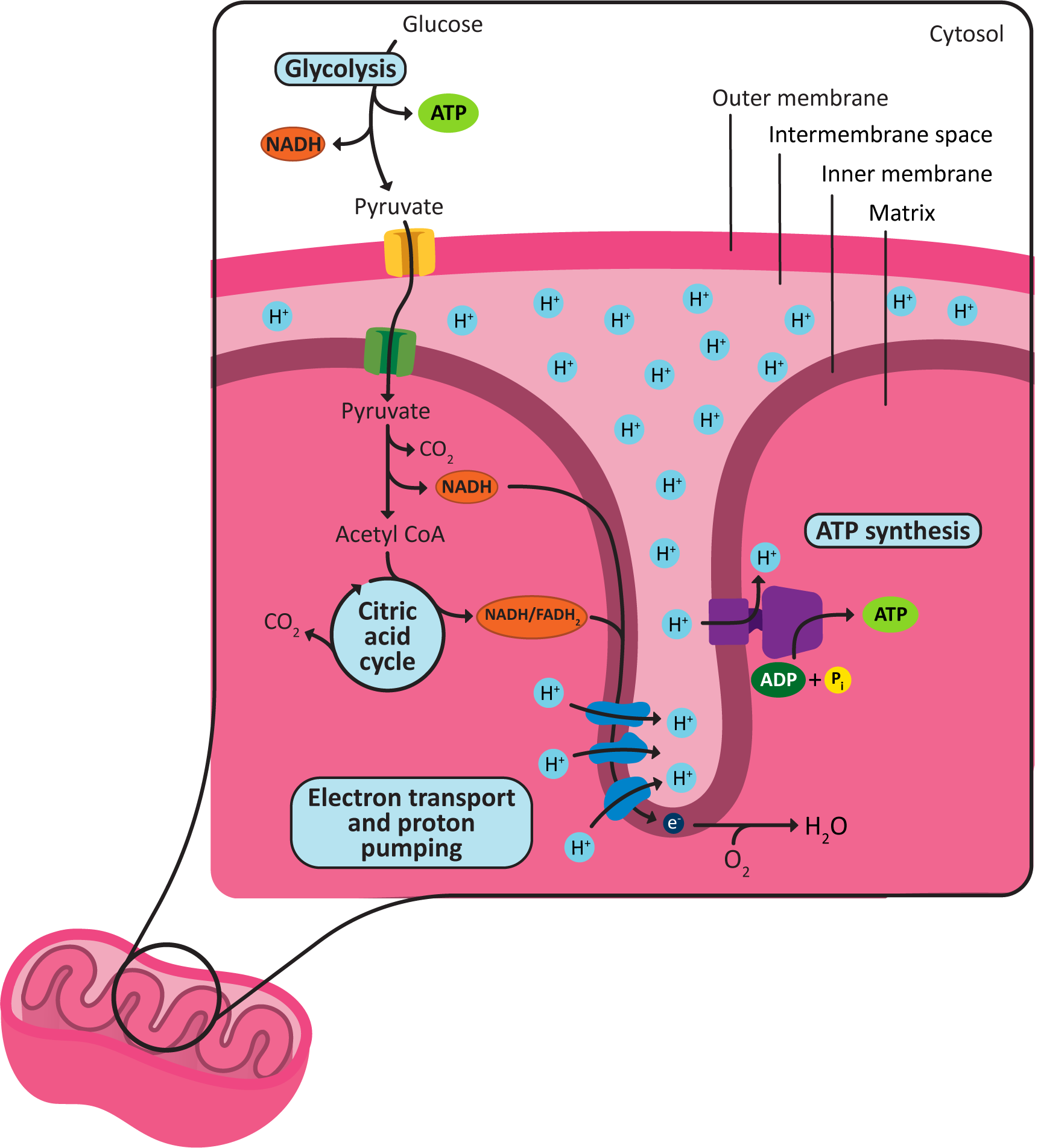
There are a couple important details you should make sure that you understand from Figure 05-13:
- The location of each of the metabolic steps of cellular respiration (glycolysis, Acetyl-CoA synthesis, the citric acid cycle, and the ETC and ATP synthesis), including which membrane they are attached to (if any).
- The areas of low and high pH based on the location of the protons in the drawing. Also note where the protons flow (from which structure to which structure) during the process of oxidative phosphorylation.
It is also worth noting where and how the chemiosmotic coupling reactions take place:
- For example, despite the fact that the ATP synthase is embedded in the cristae, we say that ATP production occurs in the matrix, as the active site is located on the matrix side of the membrane, and neither ATP nor its original reactants cross the membrane during the catalyzed reaction.
- On the other hand, we say the gradient is formed across the inner membrane, as the proteins that do the work transfer the proton from one side of the inner membrane/cristae to the other.
The video below provides some additional information, based on molecular data, about how the mitochondria may function. Not only does it show how the ETC and ATP synthase works, but it also starts with an animation of a protein being imported into the mitochondrial matrix via the TOM/TIM transporters.
Other Functions of the Mitochondrion
In introductory textbooks such as this, it is very common to focus almost exclusively on the role the mitochondrion plays in cellular respiration, as this is obviously a very important function that is key to our survival. However, that is not the only function that is carried out by this organelle. It has a number of vital roles that it plays in cellular function. Some of these functions include the following:
- It can help regulate broader cellular processes due to its ability to control access to ATP and energy. Events such as when cell division will take place appear to be linked to a functional mitochondrion actively producing ATP via oxidative phosphorylation.
- It acts as a storage site for calcium ions. Calcium is an important signaling molecule (which will be discussed in Chapter 7). As a result, the mitochondrion plays a key role in several signaling pathways involving calcium.
- It generates heat. This is done at the expense of ATP production, as it runs the electron transport chain, but the ions are allowed to return to the matrix without harnessing the energy in ATP. When the cell does this, we say that it has uncoupled the ETC from the ATP synthase. This is very useful for young infants, who experience more heat loss due to their small size. Running the ETC in this way happens in a type of fat known as brown fat, and it helps keep the baby warm. It is also used by many plants for early spring flowering (to break through the snow) and to attract pollinators.
- It helps with the production of steroids, such as cholesterol, testosterone, and other hormones.
- It is involved in the regulation of programmed cell death, a process in which a cell within a multicellular organism intentionally dies in response to specific cues. This is important in many cellular processes and also in getting rid of severely damaged cells. A good example is the loss of cells connecting the fingers during embryonic development in humans and many other animals. The “webbing” must all be removed, so the cells in this area intentionally die off, and the fingers are released from each other.
The Chloroplast
Chloroplasts are part of a larger family of organelles in plants known as plastids (Figure 05-13). There are several different types of plastids in plants, most of which have no photosynthetic activity. They are involved in a variety of functions, including storage; organ coloration; production of sugars, lipids, and nucleotides; and even gravity sensing in roots and shoots.
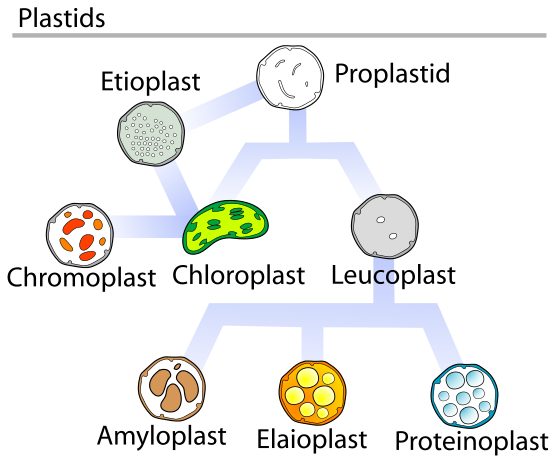
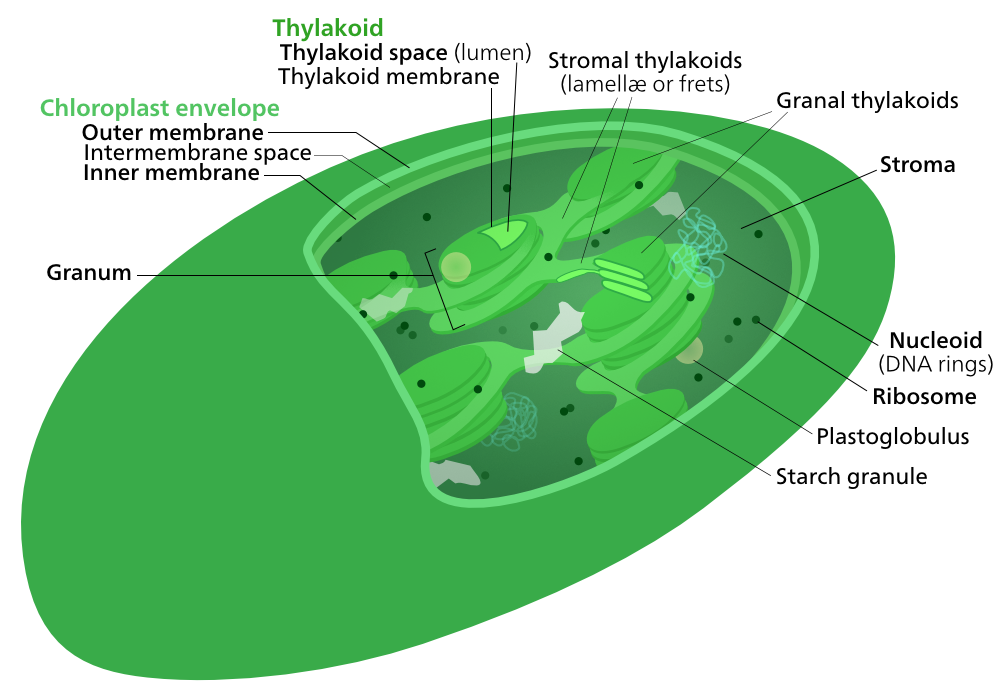
In many ways, chloroplasts have a similar structure to mitochondria in that they have an inner and an outer membrane (compare Figures 05-10 and 05-15). However, they also have a third internal membrane system not present in mitochondria, known as the thylakoid membrane. Inside this membrane is a compartment known as the thylakoid lumen. Figure 05-15 shows the chloroplast of a flowering plant, which we can tell because of the organization of the thylakoid into grana (that look like stacks of coins). Other photosynthetic organisms (most notably the many different kinds of algae) will have a slightly different organization of their flattened thylakoid sacs.
In addition to being the site of photosynthesis, resulting in the production of large amounts of sugars, chloroplasts have other roles as well. Some of these functions will result in the production of material that must be stored in the stroma of the thylakoid for a time. In Figure 05-15 above, you can see this in the starch granules (storing glucose) and plastoglobules (storing lipids and proteins) that can be observed in the stroma of the organelle.
Chloroplast Function
Photosynthesis and the Production of Sugars
Of course, the most famous function of the chloroplast is the process known as photosynthesis. In other words, the chloroplast is the site where light energy is captured and used to transform CO2 into carbohydrates. Much like our approach when discussing the mitochondrion, here we are going to focus on understanding how the structure of the chloroplast facilitates its ability to do photosynthesis.
Chloroplasts must maintain a process that
- captures light energy, which is then used to…
- produce a proton gradient to drive the production of ATP and NADPH, and then…
- fix atmospheric carbon dioxide, reduce it, and turn it into carbohydrates that the cell can use.
These processes occur in different parts of the chloroplast. The light-dependent reactions are the ones where the energy of the sun is captured and then converted into chemical energy the chloroplast can use. The light reactions include the chloroplast electron transport chain and the ATP synthase. Just like in the mitochondrion, both are membrane bound. In the chloroplast, both are embedded in the thylakoid membrane and pump protons back and forth from the stroma, to the thylakoid lumen, and then back again. The chemical energy produced by the light-dependent reactions (i.e., ATP and another electron carrier known as NADPH) will be fed into the next part of the process, called the carbon-fixation reactions (also known as the light-independent reactions, or the Calvin cycle). Most of the enzymes involved in carbon fixation are soluble and pack the stroma. The product of carbon fixation is sugars, which then can be used in a number of ways in the plant cell (energy storage, plant cell wall synthesis, etc.).
Figure 05-16, below, helps illustrate where everything happens.
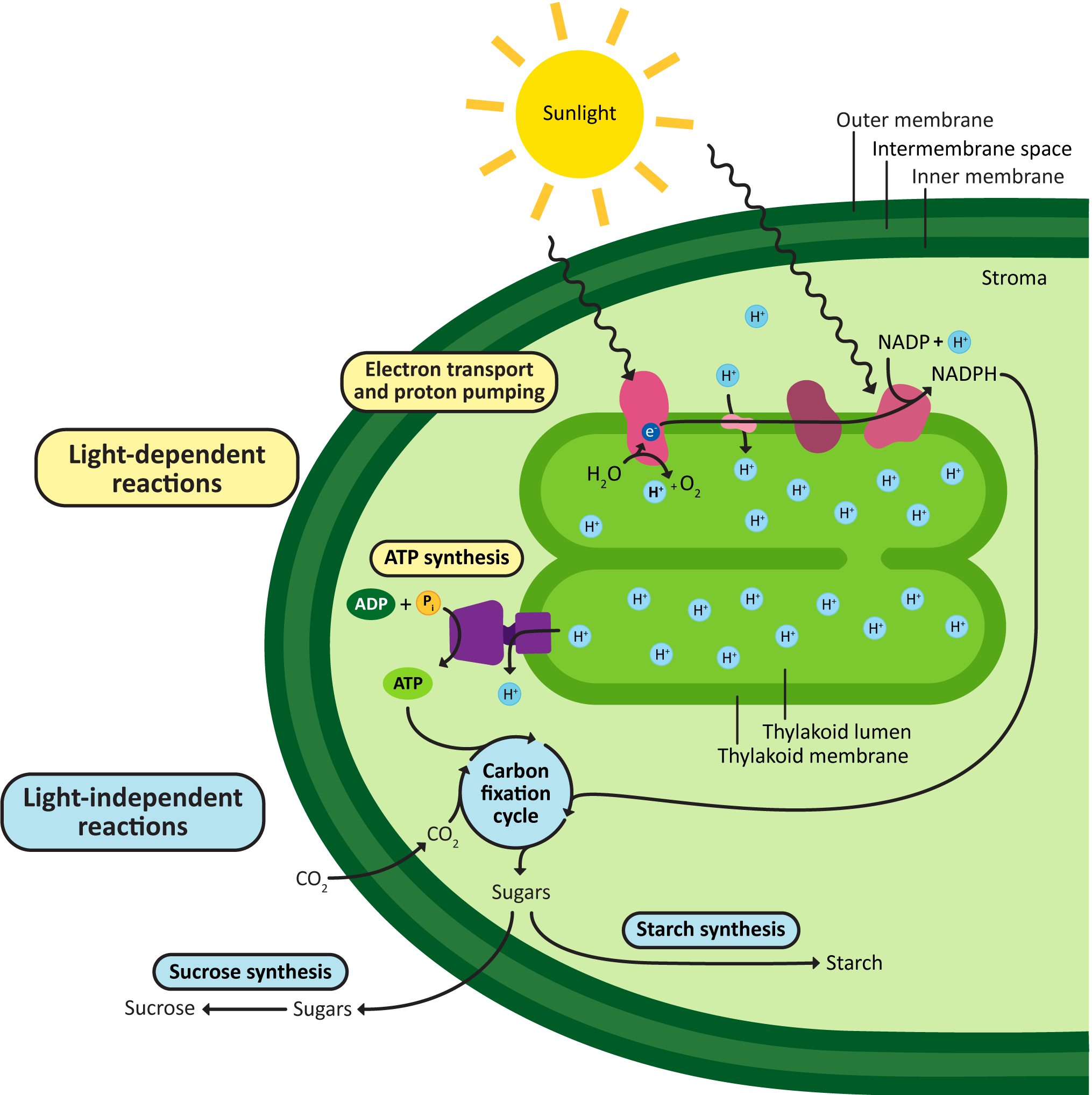
Once again, it’s important to take time to really understand Figure 05-16. Consider carefully how this compares to the organization of mitochondrion and cellular respiration, as seen in Figure 05-12. Think again about where the areas of high and low pH would be, the direction of protein flow across the ETC, and the site of ATP synthesis.
A few other things to note:
- The goal of photosynthesis in the chloroplast is to produce and export sugars. ATP is made in an intermediate step but then used up by the synthesis of the sugars.
- While the chloroplast can synthesize ATP, it cannot export it. The ATP is entirely used up in the process of carbohydrate production.
- The plant’s mitochondria must still be used to supply ATP to the rest of the plant cell.
Other Roles of the Chloroplast
Like the mitochondria, the chloroplast has additional important functions beyond photosynthesis. As autotrophs, plants must make every molecule that they need to survive, and the chloroplast plays a key role in the synthesis of many of these essential compounds, including the following:
- Almost all of the amino acids for the plant cell are synthesized by the chloroplast. The only exception is the sulfur-containing amino acids, cysteine and methionine, which are made elsewhere in the cell.
- The purines and pyrimidines used to produce nucleic acids are also synthesized here.
- It is involved (along with the ER) in the production of complex lipids, such as fatty acids and some sterols.
- It is involved in the production of stress response chemicals that are involved in triggering the plant immune response.
Chapter Summary
In this chapter, we have taken a deep dive into the structure and function of the mitochondria and chloroplasts. First, we explored their evolutionary origins, which are described by the endosymbiont theory. Their evolutionary origins explain quite a bit of their structure and how similar it is to their closest living bacterial relatives. We also looked at how proteins that are translated in the cytosol are able to be imported into either the mitochondrion or the chloroplast (but not both).
We focused heavily on the similar purpose of these organelles, in that they both create chemical energy for the cell, but the products they create are different. Mitochondria focus on creating ATP, which can be exported and used in the rest of the cell, whereas chloroplasts do not export any of the ATP that they make. Instead, they use the ATP to create carbohydrates, which are then exported and used by the cell in a variety of ways.
Finally, we explored the structure of each of the organelles as well as the additional roles that these organelles play in the proper function of the cell.
Review Questions
Note on usage of these questions: Some of these questions are designed to help you tease out important information within the text. Others are there to help you go beyond the text and begin to practice important skills that are required to be a successful cell biologist. We recommend using them as part of your study routine. We have found them to be especially useful as talking points to work through in group study sessions.
Topic 5.1: Evolutionary Origins and Protein Targeting
- Shown below are a few major features of mitochondrial and chloroplast structure and function. Explain how the features of these elements are consistent with the notion that they originated from prokaryotes:
- cell-surface membranes
- internal membranes
- ribosomes
- genomes
- electron transport systems
- formation of ATP
- any other characteristics that you can think of
- What features are shared by mitochondria, chloroplasts, and bacteria that are not shared by their eukaryotic hosts?
- Compare and contrast the mechanisms of import into mitochondria and chloroplasts with import into the other organelles that were discussed in previous topics.
Topic 5.2: Function of Mitochondria and Chloroplasts
- What is the main energy-storing molecule produced by mitochondria? Chloroplasts?
- Compare the nature of the electrochemical gradient involved in the generation of ATP in mitochondria with that in chloroplasts.
- Compare and contrast mitochondria and chloroplasts with regard to structure and function.
- List the main products of the mitochondrial electron-transfer reactions.
- List the main products of the photosynthetic electron-transfer reactions. Why is light needed for these reactions?
- The conversion of CO2 to carbohydrates does not directly require light energy. What necessary components does light energy indirectly provide for this conversion to occur?
- If chloroplasts make ATP and synthesize sugars, then why do plants need mitochondria even when growing in the light?
- Trace a molecule of oxygen from its entry into an organism, through the cell, and into the mitochondria for use in oxidative phosphorylation.
- Trace energy from the sun to energy in ATP in the cytosolic compartment of a plant cell.
Topic 5.3: Structure-Function Relationships in Mitochondria and Chloroplasts
- Make a diagram of a chloroplast and a mitochondrion and label the diagram(s) with the following terms and structures. Note that all structures are not expected to be in both organelles.
- outer, inner, and thylakoid membranes and cristae
- matrix, stroma, thylakoid lumen, and intermembrane space
- relative pH of each compartment
- ATP synthase and compartment where ATP synthesis occurs
- mitochondrial electron transport chain
- light-dependent reactions
- carbon-fixation reactions
- citric acid cycle enzymes
- site of organelle DNA and ribosomes
- pyruvate transferase
- ATP exporter
- porins
- protein import machinery (i.e., TIM/TOM, TIC/TOC)
- Both the mitochondrion and the chloroplast have several other functions that are not directly related to energy production… What are they?
References
Bölter, B., & Soll, J. (2016). Once upon a time—chloroplast protein import research from infancy to future challenges. Molecular Plant, 9(6), 798–812. https://doi.org/10.1016/j.molp.2016.04.014
Wiedemann, N., & Pfanner, N. (2017). Mitochondrial machineries for protein import and assembly. Annual Review of Biochemistry, 86(1), 685–714. https://doi.org/10.1146/annurev-biochem-060815-014352
“The leading evolutionary theory of the origin of eukaryotic cells from prokaryotic organisms. The theory holds that mitochondria, plastids such as chloroplasts, and possibly other organelles of eukaryotic cells are descended from formerly free-living prokaryotes (more closely related to the Bacteria than to the Archaea) taken one inside the other in endosymbiosis.” Source: Symbiogenesis. (2023, May 28). In Wikipedia, The Free Encyclopedia. https://en.wikipedia.org/wiki/Symbiogenesis
A type of asexual reproduction where an organism divides into two of the same.
A protein in the outer membrane of chloroplasts and mitochondria that allows small molecules to pass through via diffusion.
“Involves the engulfment of a cell by another free living organism.” Source: Symbiogenesis. (2023, May 28). In Wikipedia, The Free Encyclopedia. https://en.wikipedia.org/wiki/Symbiogenesis
“Occurs when the product of primary endosymbiosis is itself engulfed and retained by another free living eukaryote.” Source: Symbiogenesis. (2023, May 28). In Wikipedia, The Free Encyclopedia. https://en.wikipedia.org/wiki/Symbiogenesis
The mitochondria and chloroplast targeting signal. It is an N-terminal sequence that forms a helical structure needed for correct receptor recognition and transport to the proper organelle.
Part of the TIM/TOM complex, TOM (transporter outer mitochondrial membrane) lives in the outer mitochondrial membrane to help facilitate mitochondrial proteins’ transfer from the cytoplasm into the mitochondrial matrix.
Part of the TIM/TOM complex, TIM (transporter inner mitochondrial membrane) lives in the inner mitochondrial membrane to help facilitate mitochondrial proteins’ transfer from the cytoplasm into the mitochondrial matrix.
“An enzyme complex found in mitochondria which cleaves signal sequences from mitochondrial proteins.” Source: Mitochondrial processing peptidase. (2023, January 29). In Wikipedia, The Free Encyclopedia. https://en.wikipedia.org/wiki/Mitochondrial_processing_peptidase
An N-terminal region of amino acids that serves as the chloroplast targeting sequence.
Part of the TIC/TOC complex, TOC (transporter outer chloroplast membrane) lives in the outer chloroplast membrane to help facilitate chloroplast proteins’ transfer from the cytoplasm into the chloroplast stroma.
Part of the TIC/TOC complex, TIC (transporter inner chloroplast membrane) lives in the inner chloroplast membrane to help facilitate chloroplast proteins’ transfer from the cytoplasm into the chloroplast stroma.
A chloroplast enzyme that cleaves off the transit peptide of proteins in the stroma of chloroplasts.
“A metabolism reaction that results in the production of ATP or GTP supported by the energy released from another high-energy bond that leads to phosphorylation of ADP or GDP to ATP or GTP.” Source: Substrate-level phosphorylation. (2023, March 13). In Wikipedia, The Free Encyclopedia. https://en.wikipedia.org/wiki/Substrate-level_phosphorylation
Refers to the cellular mechanism that harnesses the potential energy stored in a chemical gradient to power a biochemical reaction.
“The metabolic pathway in which cells use enzymes to oxidize nutrients, thereby releasing chemical energy in order to produce adenosine triphosphate (ATP).” Source: Oxidative phosphorylation. (2023, July 17). In Wikipedia, The Free Encyclopedia. https://en.wikipedia.org/wiki/Oxidative_phosphorylation
The biochemical process of turning light energy into ATP.
A protein that moved protons from one side of the membrane to the other, creating a gradient.
“A gradient of electrochemical potential, usually for an ion that can move across a membrane. The gradient consists of two parts: 1) The chemical gradient, or difference in solute concentration across a membrane. 2) The electrical gradient, or difference in charge across a membrane.” Source: Electrochemical gradient. (2023, July 18). In Wikipedia, The Free Encyclopedia. https://en.wikipedia.org/wiki/Electrochemical_gradient
“A series of protein complexes and other molecules that transfer electrons from electron donors to electron acceptors via redox reactions (both reduction and oxidation occurring simultaneously) and couples this electron transfer with the transfer of protons (H+ ions) across a membrane.” Source: Electron transport chain. (2023, May 19). In Wikipedia, The Free Encyclopedia. https://en.wikipedia.org/wiki/Electron_transport_chain
A series of biochemical reactions that produces ATP and the electron donors NADH and FADH2 from pyruvate. Also known as the tricarboxylic acid (TCA) cycle.
A large protein complex that uses the flow of protons to synthesize ATP from ADP and inorganic phosphate. It dimerizes and is found in the cristae membrane of mitochondria.
A description of the potential energy captures in a proton gradient. When the protons are allowed to flow down their gradient through ATP synthase, this allows for ATP to be made much like a hydroelectric dam makes electricity from the flow of water through turbines.
An organism that creates its own complex biomolecules (carbohydrates, proteins, lipids) from simple substrates (carbon dioxide and water).
Autotrophic organisms that provide the base of the food chain.
“An organism that cannot produce its own food, instead taking nutrition from other sources of organic carbon, mainly plant or animal matter.” Source: Heterotroph. (2023, February 19). In Wikipedia, The Free Encyclopedia. https://en.wikipedia.org/wiki/Heterotroph
A membrane-bound organelle in a cell known to produce and secrete a chemical currency called ATP.
The area inside the mitochondria where the TCA cycle, mitochondrial DNA, and mitochondrial ribosomes are located.
“A fold in the inner membrane of a mitochondrion. The name is from the Latin for crest or plume, and it gives the inner membrane its characteristic wrinkled shape, providing a large amount of surface area for chemical reactions to occur on.” Source: Crista. (2022, November 5). In Wikipedia, The Free Encyclopedia. https://en.wikipedia.org/wiki/Crista
“The metabolic pathway that converts glucose (C6H12O6) into pyruvate.” Source: Glycolysis. (2023, July 23). In Wikipedia, The Free Encyclopedia. https://en.wikipedia.org/wiki/Glycolysis
“A chemical entity that donates electrons to another compound. It is a reducing agent that, by virtue of its donating electrons, is itself oxidized in the process.” Source: Electron donor. (2022, August 19). In Wikipedia, The Free Encyclopedia. https://en.wikipedia.org/wiki/Electron_donor
A membrane-bound organelle found in plants. Commonly plastids will differentiate into chloroplasts, but other examples include leucoplasts and chromoplasts.
“The thylakoid membrane is the site of the light-dependent reactions of photosynthesis with the photosynthetic pigments embedded directly in the membrane.” Source: Thylakoid. (2023, May 24). In Wikipedia, The Free Encyclopedia. https://en.wikipedia.org/wiki/Thylakoid
“The thylakoid lumen is a continuous aqueous phase enclosed by the thylakoid membrane. It plays an important role for photophosphorylation during photosynthesis.” Source: Thylakoid. (2023, May 24). In Wikipedia, The Free Encyclopedia. https://en.wikipedia.org/wiki/Thylakoid
“Chloroplast thylakoids frequently form stacks of disks referred to as grana (singular: granum).” Source: Thylakoid. (2023, May 24). In Wikipedia, The Free Encyclopedia. https://en.wikipedia.org/wiki/Thylakoid
“Storage areas where starch molecules are stored at night.” Source: Starch. (2023, July 25). In Wikipedia, The Free Encyclopedia. https://en.wikipedia.org/wiki/Starch
“Spherical bubbles of lipids and proteins about 45–60 nanometers across. They are surrounded by a lipid monolayer. Plastoglobuli are found in all chloroplasts.” Source: Chloroplast. (2023, July 14). In Wikipedia, The Free Encyclopedia. https://en.wikipedia.org/wiki/Chloroplast
The area inside the chloroplast inner membrane that surrounds the thylakoids.
The biochemical reactions that occur in the plant chloroplast that depend on light. These include the process of photophosphorylation.
The biochemical reactions that occur in the chloroplast stroma, where carbon dioxide is used to make three carbon sugar molecules.