6 The Cytoskeleton
Introduction
We often think of the many pathways of the cytoskeleton as “the highways of the cell,” yet the reality is so much more complex and fascinating. They do not simply act as highways but highways that can disassemble and completely rebuild themselves when the need arises. Throughout the previous chapters of this book, we have made reference to the cytoskeleton and its role in the various cellular processes. This is because the cytoskeleton is involved in virtually every aspect of cellular function. It’s impossible to ignore the central role the cytoskeleton plays in the cell. The cytoskeletal “highways” help provide structure and connection within the cell and between cells. The cytoskeleton influences the shape of the cell and aids in the relay of signals from one part of the cell to another. The cytoskeleton helps cells grow, move, retract, and even die. We can see the impact of the cytoskeleton in every chapter you’ve read thus far, and it will continue to play an important role in the remaining chapters.
In this chapter, we will explore the components of the cytoskeleton, the details of how it is built, and how it functions. We will highlight some specific examples of function that are considered more universal, but know that there are so many more examples that could also be included.
Topic 6.1: Overview of the Cytoskeleton and Intermediate Filaments
Learning Goals
- Compare and contrast the structure and function of the three types of cytoskeletal elements: actin filaments, intermediate filaments, and microtubules.
- Identify the different types of cytoskeletal elements in micrographs.
- Discuss different types of intermediate filaments, and correlate their structure with the strength of the assembled, functional cytoskeletal element.
Introduction
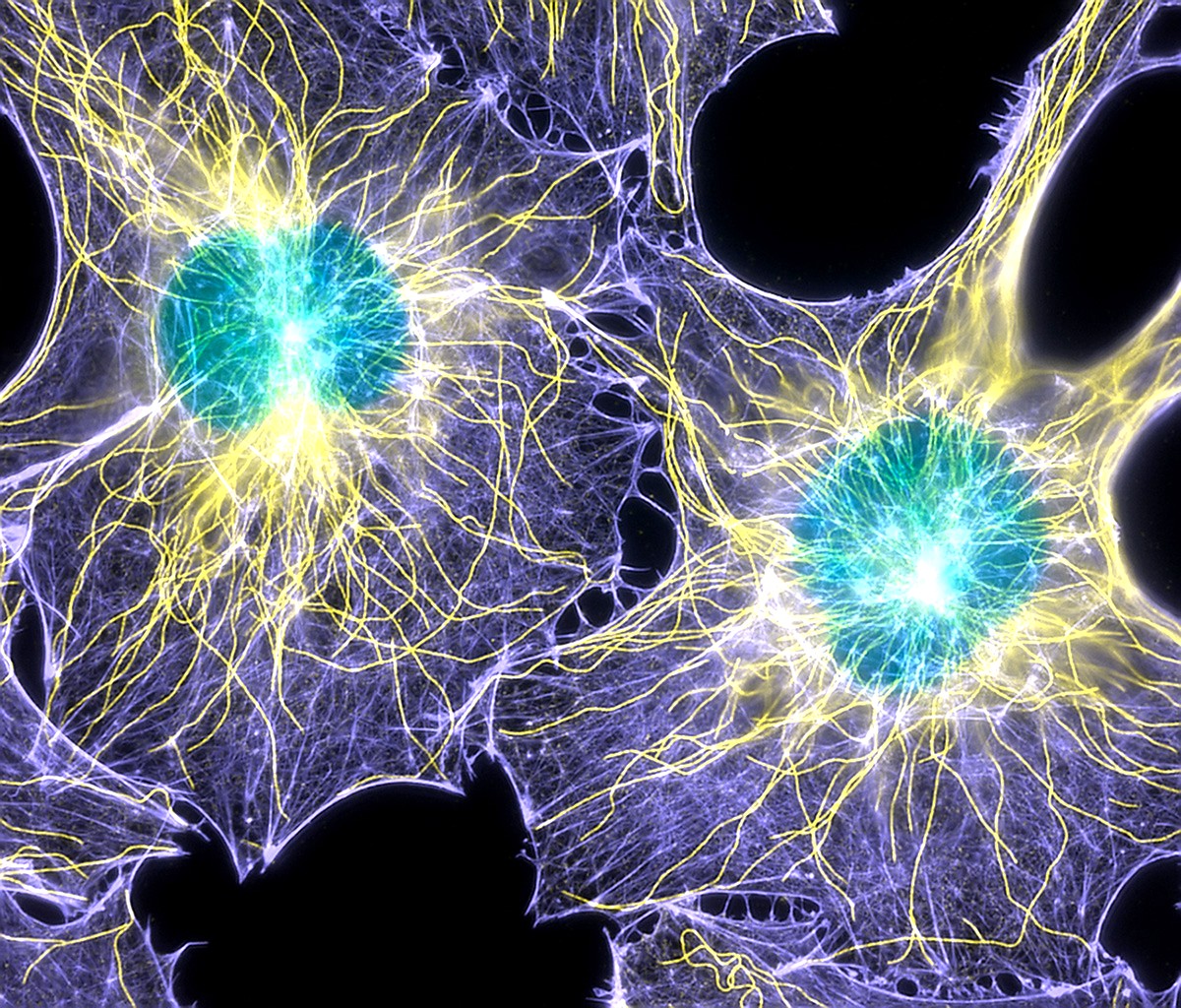
The cytoskeleton is a filamentous network that extends throughout the cell. There are three different types of cytoskeletal filament that exist. All known eukaryotic cells have both actin filaments and microtubules (Figure 06-01). The third type, intermediate filaments (Figure 06-02), is no less important in the cells that have them; however, they are really only found in the cells of vertebrates. Collectively, all three of these filaments have vital roles to play in virtually every function in the cell. Not only do they help give the cell its shape, but they also help resist mechanical stresses; act as an anchor for many proteins and organelles; provide tracks for transport of vesicles, organelles, and other cargo; and aid in the locomotion of many cell types. They also help with the division of both the cytoplasm and the DNA during mitosis.
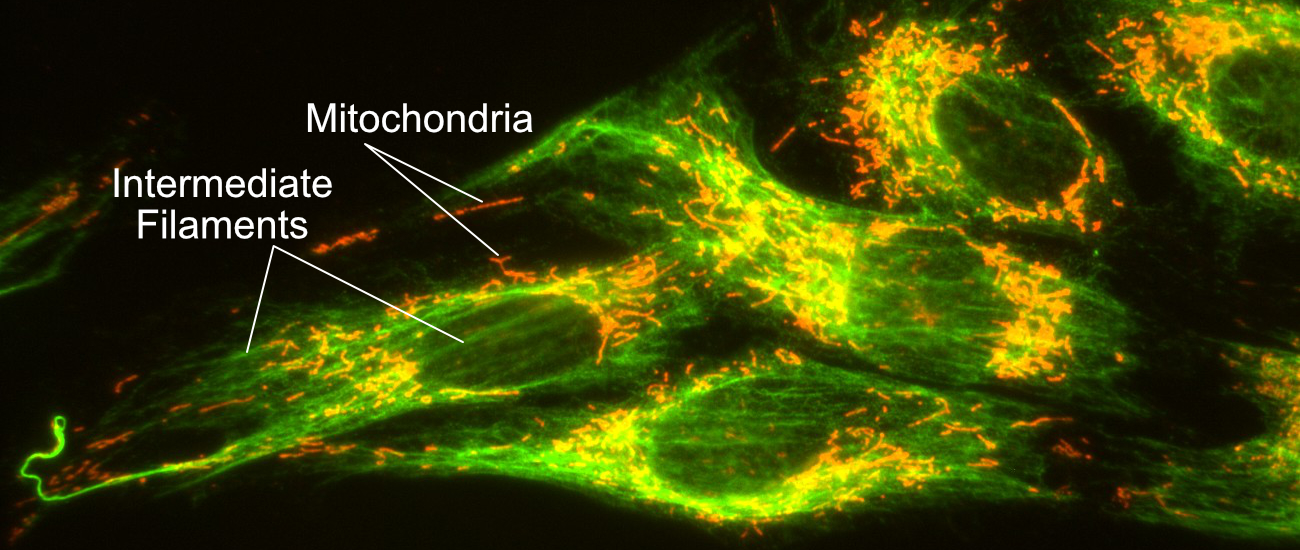
Even cell types that were previously thought to lack a cytoskeleton have now been shown to have proteins that fulfill these roles. A very good example of this are bacteria, which were originally thought to have no cytoskeleton at all, but we now know have a cytoskeleton-like network that functions similarly. Another example is plant and insect cells, which do not have intermediate filament genes in their genomes, yet they both still have a nuclear lamina. To build it, they use genetically unrelated proteins, which perform the function of nuclear lamins (we call them lamin-like proteins). As such, it is safe to say that the cytoskeleton is (another) essential component of all living cells. Cells simply cannot survive without it.
The features of each of the types of cytoskeletal filaments that we list here will be explained in further detail throughout the rest of the chapter. For now, here is a quick summary, in order of filament size (see also Video 06-01):
- Microtubules (MT)—described in Topic 6.2
- The largest of the three types (~25 nm in diameter).
- Made of repeating subunits called tubulin. Tubulin itself is a dimer, made of two smaller subunits, called alpha- and beta-tubulin.
- Go through rounds of polymerization and depolymerization (dynamic instability).
- Involved in a variety of cell functions.
- In all cells, they are involved in mitosis, cargo trafficking, and maintenance of cell shape.
- In animal cells, they radiate outward from the center of the cell and act as “highways” for vesicular transport.
- In plants and algae, they play an important role in the secretion of cellulose, which happens at the plasma membrane. Thus, they are found in the cell cortex in plants (meaning that the microtubules are found directly underneath the plasma membrane).
- In fungi and other protists, the arrangement of the cytoskeleton is more variable.
- Both cilia (singular cilium) and flagella (singular flagellum) are specialized structures that depend on microtubules for function.
- Intermediate filaments (IF)—described later in this topic (6.1)
- Their diameter is “in between” the other two types of cytoskeletal filaments (~10 nm).
- They are a family of proteins classified into five major types (e.g., keratins, vimentin, neurofilaments, and nuclear lamins). These types describe the subunit that they are made from.
- Generally, only one or two subtypes are expressed in each cell types (i.e., nuclear lamins plus keratin, for example).
- A single intermediate filament is always made from the same kind of subunit. They do not mix and match.
- They do not undergo dynamic instability, but they are assembled and disassembled as needed.
- They help with anchoring cells that are not motile, resist mechanical strain in tissues, and are vital to the maintenance of cell shape. Nuclear lamins are very important in the maintenance and organization of the nucleus.
- Actin filaments (AF)—described in Topic 6.3
- Also known as microfilaments.
- The smallest of the three types (~7 nm in diameter).
- Made of repeating subunits, also called actin.
- Monomers of actin are sometimes referred to as g-actin to differentiate from the filament form, which is call f-actin.
- Like microtubules, they also undergo dynamic instability.
- In animal cells, they are heavily involved in cellular locomotion but also cellular adhesion and shape, cytokinesis, cargo trafficking, and muscle contraction. As a result, actin filaments tend to remain close to the plasma membrane, in the cell cortex.
- In plant and algae cells, the cellular arrangement is different. They are found throughout the cytoplasm (not just underneath the plasma membrane). As such, they are involved more heavily in cargo trafficking and maintenance of cell shape (plant cells are not motile). They also help produce the phenomenon known as cytoplasmic streaming.
- In other cells, once again, the arrangement is more variable.
Intermediate Filaments (IFs)
As mentioned, intermediate filaments get their name from the fact that they are “intermediate” in size between actin and microtubules. Their importance in the cell is often overshadowed by the focus on actin and microtubules when discussing the cytoskeleton and by the fact that both plants and insects do not appear to carry any genes in this family. This discovery initially implied they were “less important” than the “universally essential” filaments. However, we have since discovered that even though the genes for intermediate filaments are not always present, there are often other proteins that are able to functionally fill this role. For example, there is always a structure that resembles the nuclear lamina in all nuclei, and it functions similarly, suggesting that it plays a vital cellular role. In addition, in the cell types that contain additional intermediate filaments (like skin or neurons), they play a key role, helping to maintain shape and resist mechanical strain. Thus, intermediate filaments, or intermediate filament-like proteins, are an essential cytoskeletal element for all cells.
Intermediate filaments (IFs) are a genetic family of proteins rather than a single highly conserved type. Within one filament, only one type of protein subunit is present. Each intermediate filament type is tissue specific, which means that you can tell cell type based on what kind of IF it expresses. For example, neurons express neurofilaments, but skin cells do not.
Some of the most well-known types of intermediate filaments include the following:
- Keratin: Found in epithelial cells and their derivatives, hair, nails, and horn. They help hold skin cells to each other and to the underlying membrane and provide resistance against mechanical tension (also made famous by shampoo commercials promoting their effects on keratin).
- Vimentin: Found in intracellular fibers of connective tissue cells, including muscle. They help hold tissues together and provide strength.
- Neurofilaments: Found in neurons. They help form and support the characteristic (and extreme!) shape of a nerve cell.
- Lamins: Components of the nuclear lamina. We discussed these previously in Chapter 3. They form a meshwork beneath the nuclear envelope and help maintain the integrity of the nuclear envelope.
Intermediate filaments are considered less dynamic compared to the other filaments (i.e., actin and microtubules). They do not undergo dynamic instability, which is a process of rapid switches between growth and shrinkage that is key to microtubule and actin function. However, they are still able to assemble and disassemble as the cell requires. A perfect example of this is the breakdown of the nucleus during mitosis, which is driven by the phosphorylation and breakdown of the nuclear lamina, which we saw in Chapter 3 and will discuss again later in this chapter.
Structure of Intermediate Filaments
Regardless of the specific subunit used to make it, all intermediate filaments are somewhat similar and follow the same pattern of assembly. Like all three of the cytoskeletal filaments, intermediate filaments are formed when smaller subunits, known as monomers, come together in a particular way to form a longer, filamentous polymer. So when we refer to the specific types of intermediate filament (i.e., keratin, vimentin, etc.), we are referring to the monomer being used as the building block.
All of the intermediate filament monomers are what are known as filamentous proteins, meaning that they are long and threadlike (Figure 06-03). They have a large central region that is a long alpha helix, which makes up the bulk of the filament. On either end are the “head” and “tail” region, which are specific to each of the different monomer types (keratin, vimentin, etc.). It is these head and tail regions that help the intermediate filament carry out its specific function. For example, the nuclear lamins will require sites that can bind to the chromatin, whereas keratin, which is not in the nucleus, does not require this feature.
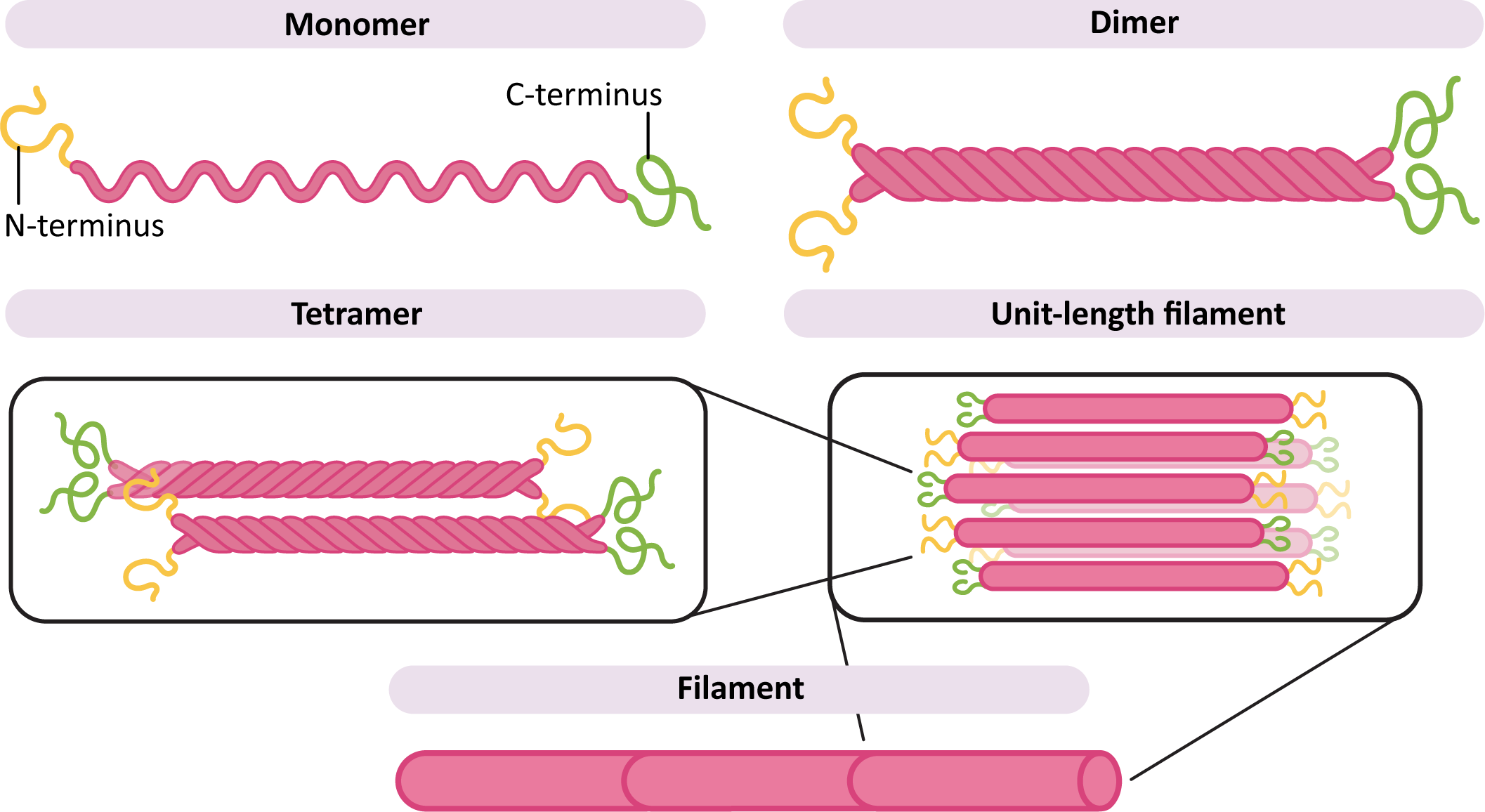
In order to build a filament (Figure 06-03), two monomers first come together to form a dimer. The dimers then assemble with each other in an antiparallel arrangement, meaning that they are oriented in the opposite direction from each other. This forms a tetramer. The tetramers then assemble to form the long filament. Since the ends of the monomers are staggered compared to each other in the filament, this forms a long, ropelike structure that doesn’t stretch and is difficult to break.
Like many protein complexes, the intermediate filament subunits undergo self-assembly in order to spontaneously form the final filament. This means that energy is not required to facilitate this assembly process (this is different from actin and microtubule assembly).
Remember from Chapter 2 that the alpha helix is a secondary structure, commonly used in protein folding. Thus, the amino acid sequence of the IF subunit is such that the alpha helix takes shape on its own. The formation of the dimers and tetramers is also facilitated by the amino acid sequence. Chemical analysis of the alpha helix shows that they have a line of nonpolar amino acids on one side of the alpha helix. This facilitates the formation of a structure known as a coiled coil (Figure 06-04). This is a very common structural strategy, as it ensures precise alignment of subunits, and the nonpolar interactions are difficult to break in the aqueous environment of the cell.
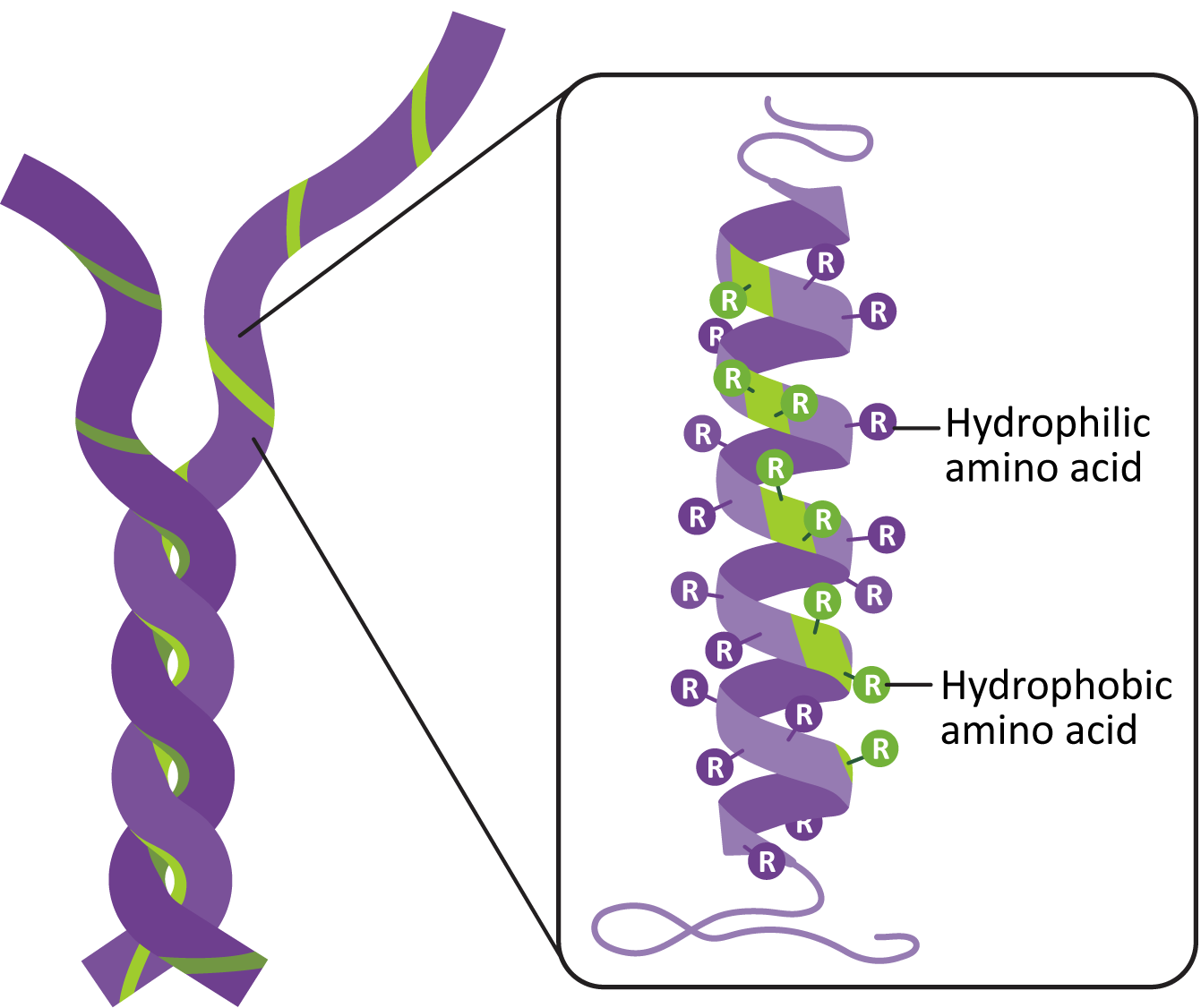
Examples of Intermediate Filaments in Cells
Example 1
The Nuclear Lamina
Since all eukaryotic cells have a nucleus, the nuclear lamina is easily the most important example of intermediate filament function. We have already discussed this function back in Chapter 3, when we examined the structure of the nucleus in detail. The nuclear lamina is vital to the proper organization and function of the nucleus throughout the cell cycle. All Eukaryotes that have a nucleus will also have a nuclear lamina of some kind.
In interphase, the assembled nuclear lamins form a latticework just underneath the nuclear envelope (Figure 06-05). Not only does this help provide structure to the nuclear envelope, but it also helps with the organization of the chromatin. The chromosomes are attached to the nuclear envelope and the underlying lamina. This attachment helps maintain the regional organization of the chromatin, thus facilitating gene expression.
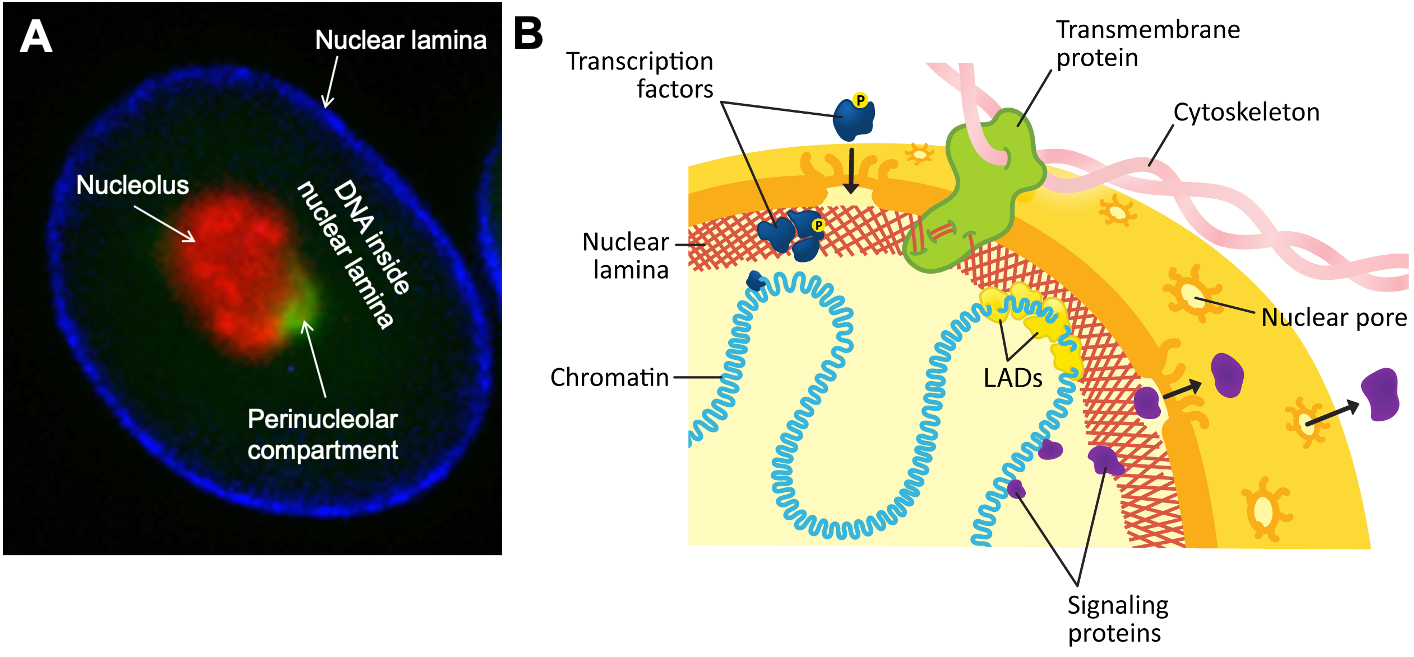
Not only is the nuclear lamina attached to the nuclear envelope and internal components of the nucleus, but it also maintains attachments to the cytoskeleton on the exterior of the nucleus (Figure 06-05). This attachment can help maintain the location of the nucleus within the cell (and allow it to be moved if necessary). A variety of proteins facilitate connections between the nuclear lamina, on the inside of the nucleus, and the cytoplasmic cytoskeleton. In some cases, specialized transmembrane proteins form complexes spanning the inner and outer membranes of the nucleus.
During mitosis, destabilization of the nuclear lamina (via phosphorylation of the lamins) is what drives the disassembly of the nuclear envelope (Figure 06-06). Once mitosis is over, the lamins are dephosphorylated, and the nuclear lamina reforms. Since it’s attached to both the membrane and the chromosomes, the result is that everything ends up back where it’s supposed to be.
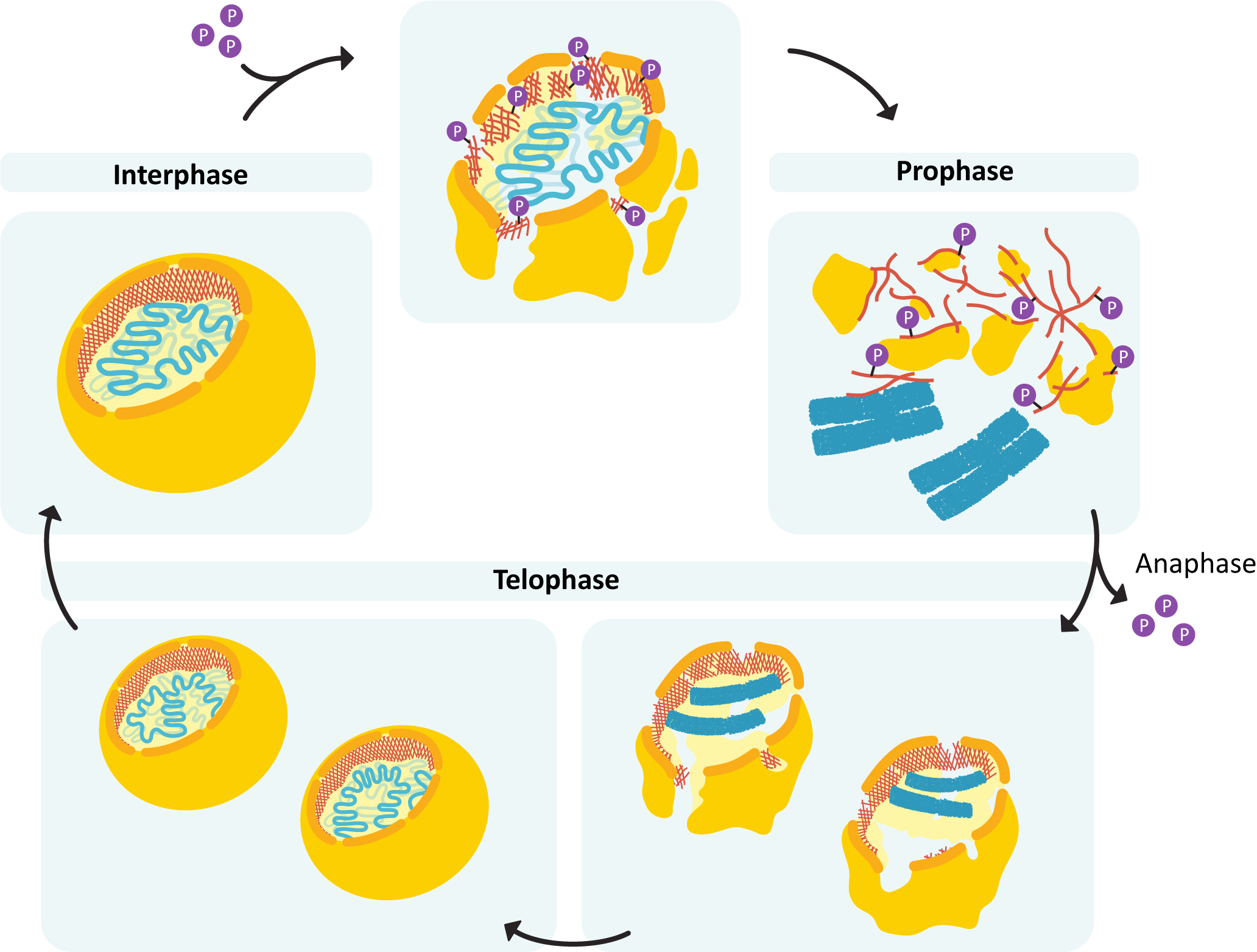
To further illustrate the importance of these nuclear lamins, here are a few examples of genetic diseases that result from mutations in lamin proteins. In one disease known as Hutchinson-Gilford progeria syndrome, children appear to age at a highly accelerated rate and often die in their early teens (Prokocimer et al., 2013). Emery-Dreifuss muscular dystrophy is caused by a few different genes, but one of them is a nuclear lamin, and others include proteins that are also involved with maintaining the shape of the nuclear envelope (Maggi et al., 2021).
Example 2
Neurofilaments and Cell Shape
As you likely know, neurons have a very distinct shape, characterized by the axon that projects out one side of the cell. Axons can be extremely long—the longest axon in the human body is the sciatic nerve, which runs from the base of your spinal cord to your big toe, so it’s about 1 m long. However, larger animals likely have much longer axons than that. (The blue whale is thought to have a dorsal root ganglion that is 25–30 m long!!!)
Cells do not easily make this kind of extreme shape…it’s a long way from the sphere that is considered to be the most energetically favorable shape for a cell! Thus, the cytoskeleton, including both microtubules and intermediate filaments, is required to maintain this structure. Neurons express a type of intermediate filament known as a neurofilament. Both neurofilaments and microtubules can be seen in the cross section of a nerve axon in Figure 06-07. If either the neurofilaments or the microtubules are disrupted, the axon will lose its integrity and retract, and the cell will die. Many kinds of dementia are the result of this kind of loss of shape due to impaired cytoskeleton function.
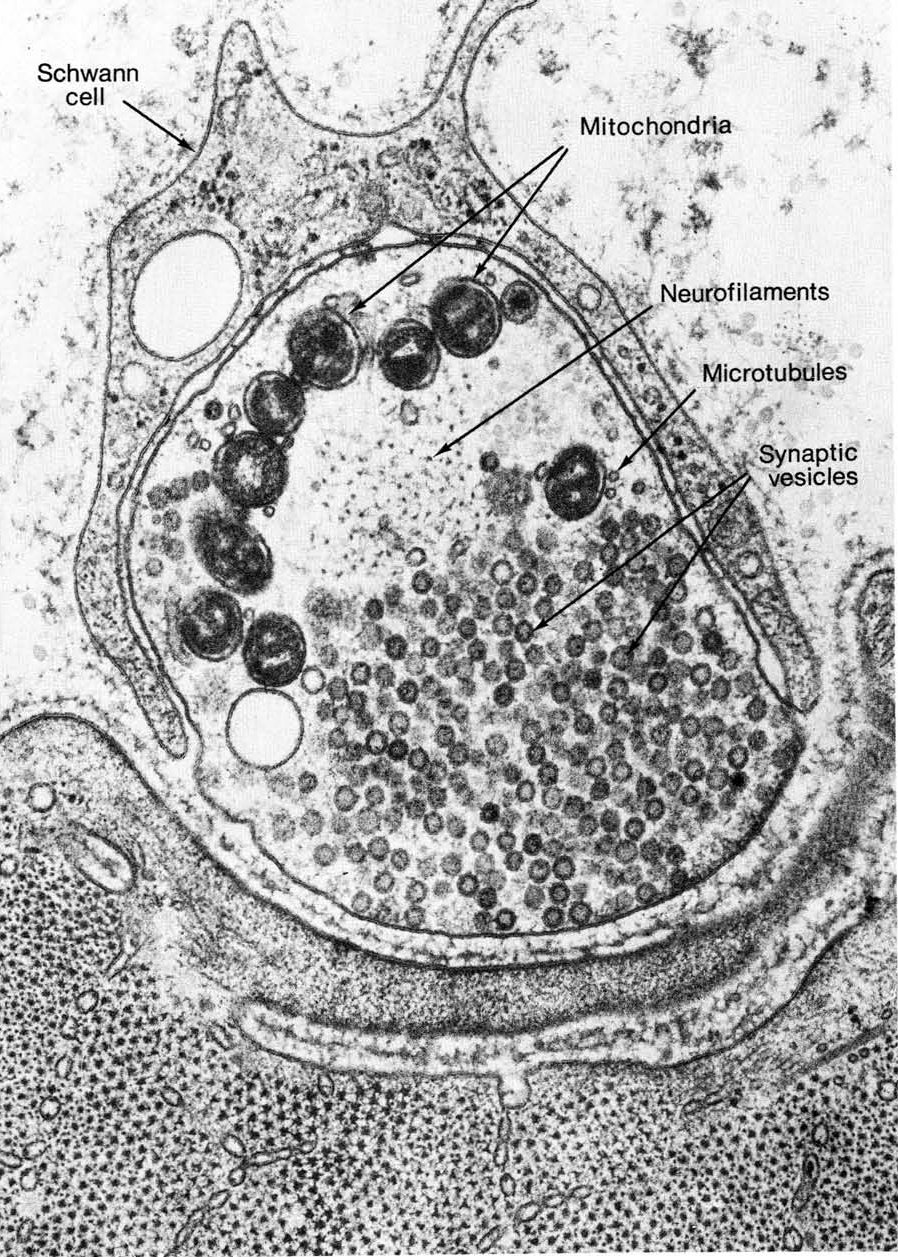
Example 3
Desmosomes and Hemidesmosomes
As was mentioned earlier, not all cells appear to require cytosolic intermediate filaments in high numbers, but in the cell types that have them, they are key to proper function. Our skin and the cells lining our gastrointestinal tract are perfect examples of this.
The role of our skin is not just to keep all of our internal bits on the inside but also to protect us from the world around us. It forms a flexible yet impenetrable barrier to pathogens, like bacteria, viruses, and other toxins. Thus, even if we twist, push, or pull on our skin, we cannot penetrate it. Breaking the skin barrier requires a sharp object that can cut through the cells and expose the tissues underneath. (We do not recommend trying this…)
In order for our skin to resist everything that we put it through, the cells need to be much stronger than what a plasma membrane can provide on its own. Intermediate filaments, specifically keratin, are used to help our skin stay strong. The keratin filaments pass from one side of the cell to the other and bind to large protein plaques in the plasma membrane. These plaques, known as desmosomes (Figure 06-08), bind to similar plaques in adjacent cells, which are attached to that cell’s keratin. The keratin + desmosome complex transmits mechanical stress along the ropes instead of through the membrane. Desmosomes act like the rivets in your jeans that hold the different pieces of fabric together. They also provide an anchor point for the keratin filaments. Not only do desmosomes attach cells to each other, but they also bind to the membrane layer underneath the skin (called the basal lamina). In this case we call them hemidesmosomes.
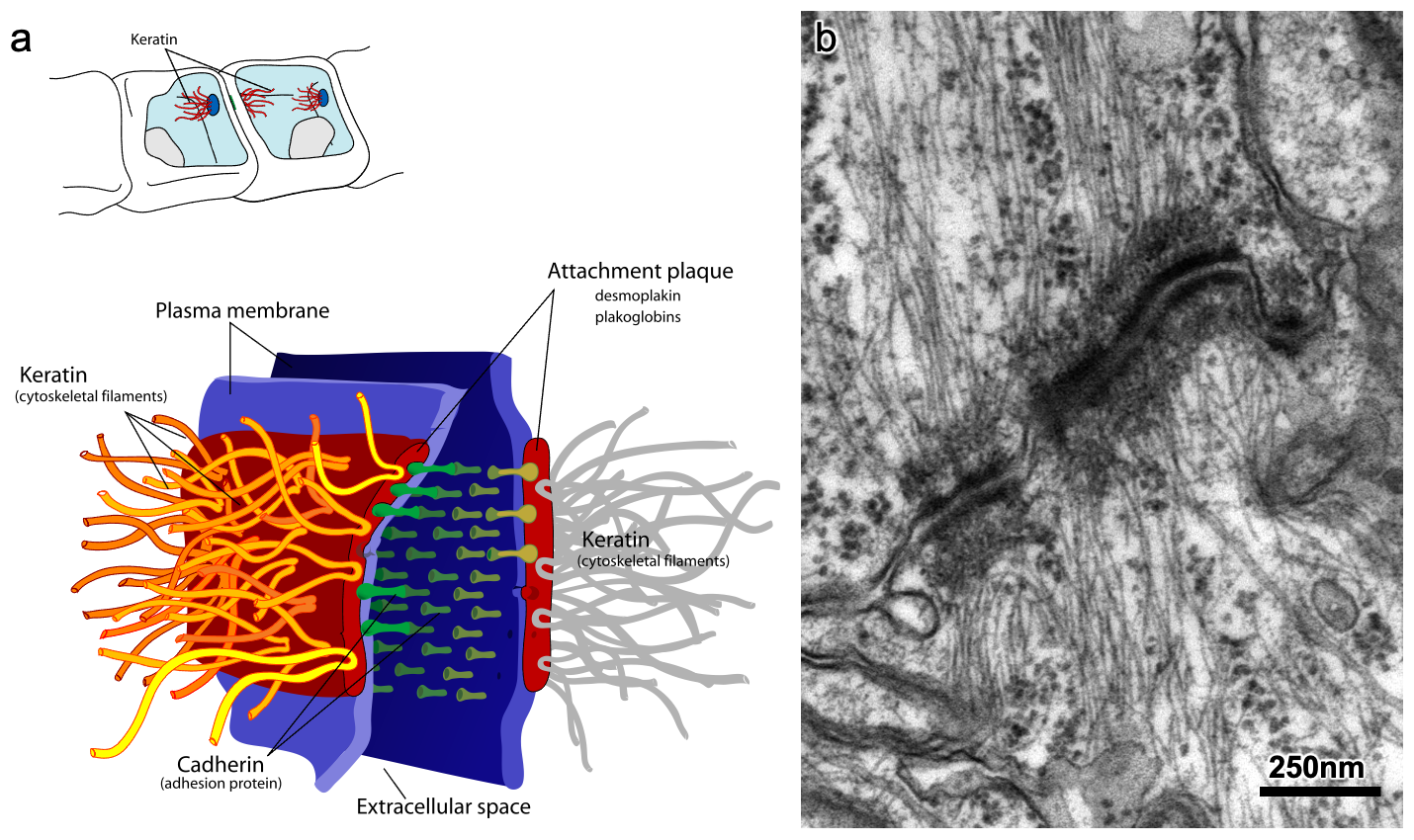
Once again, we can see the importance of intermediate filaments by examining the diseases that result from genetic mutations in keratin. Epidermolysis bullosa is a very painful disease in which the skin blisters and tears at the slightest touch. Even soft clothing and the act of eating can cause ruptures and blisters in the skin. The children born with this disease are often referred to as “butterfly children,” as their skin is incredibly fragile (like the wings of a butterfly). It is caused by several different mutations affecting the strength of the attachment of the skin, including more than one mutation in keratin genes. Like the other diseases we’ve seen in this section, there is no cure. However, regeneration of skin through stem cell therapy and grafting has shown promise in recent years.
Topic 6.2: Microtubules and Dynamic Instability
Learning Goals
- Compare the mechanics of microtubule polymerization in vivo (in a cell) and in vitro (in a test tube).
- Describe the process of dynamic instability, focusing on how tubulin dimers (a/b) interact noncovalently to make microtubules with a “plus” and “minus” end.
- Interpret live-cell images of microtubules undergoing dynamic instability and explain the current model of how GTP binding to tubulin can bring about this dynamic behavior.
- Explain how MTOCs reduce the lag phase of growing MTOC and their role in overall cell function.
- Provide examples of how accessory proteins can interact with microtubules or tubulin to influence cell function.
The second of the three types of cytoskeletal filament that we will discuss is microtubules. These filaments differ significantly from intermediate filaments in many fundamental ways. For example, their assembly and disassembly are considered more dynamic than that of intermediate filaments. Each microtubule filament is in constant flux, switching between phases of growth and shrinkage. This process of rapid growth and shrinkage is known as dynamic instability. It is fundamental to the function of both microtubules and actin filaments. As such, we will use microtubules as a case study to explore how dynamic instability works in both types of filaments. After that, we will discuss the function of microtubules prior to moving on to the final topic of this chapter, where we will discuss actin structure and function.
Microtubule Structure
Microtubules are protein polymers in the shape of hollow tubes (Figure 06-09). The tube is formed by linear associations of tubulin subunits, which we call protofilaments. There are 13 of these protofilaments arranged in a circle to form each microtubule.
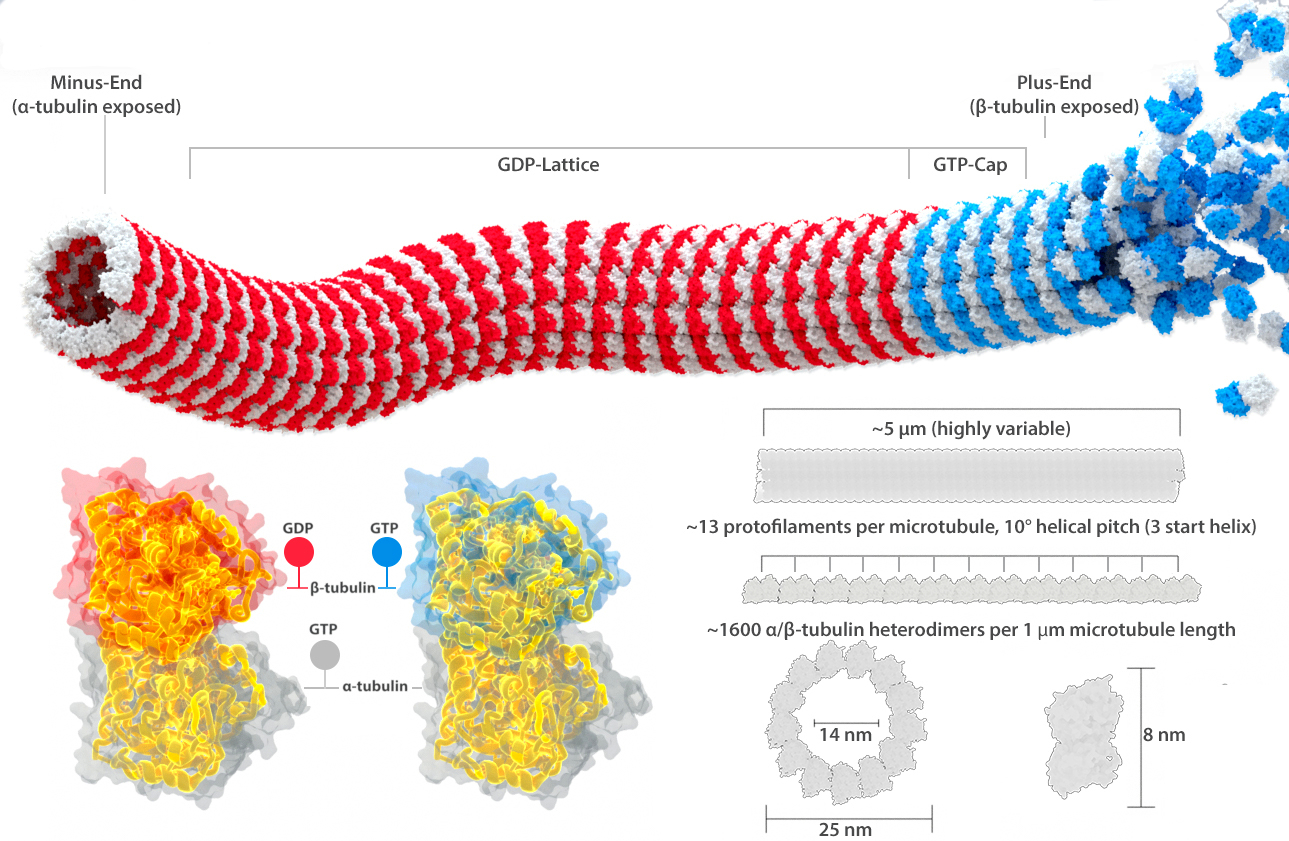
The “subunit” that is added to a growing microtubule is actually a dimer, made up of one alpha- and one beta-tubulin (Figure 06-09). Alpha- and beta-tubulin are each about 55 kDa each, and together they are about 8 nm long. The positions of tubulin dimers in the microtubule in adjacent protofilaments are slightly out of phase so that they form a spiral with a relatively low pitch, as you can see in Figure 06-09. Each monomer in the tubulin dimer also carries a GTP molecule within it. The alpha-tubulin subunit grabs the GTP and keeps it in its core but does nothing else with it. On the other hand, the GTP in the beta-subunit can undergo a hydrolysis to convert from GTP to GDP. Thus, the state of the beta-subunit, and whether it is currently bound to GTP or GDP, will affect the chemical properties of the entire tubulin dimer. This shift in chemical composition affects how well the protein monomers assemble into a polymer as well as the stability of the assembled polymer.
Before we go further, we need to take a step back and address the name of these monomers. First they are confusing. The tubulin dimer is made of a couple of different tubulin monomers, and both the monomers and the dimers all get called “tubulin” at times. For the most part, we try to refer to the dimer subunits by their actual names (alpha- and beta-tubulin, or alpha- and beta-subunit) so that when we refer to tubulin without mentioning alpha- or beta- (or gamma-, as there are even more types of tubulin that you will learn about later), you will know that this is intended to refer to the tubulin dimer. We also very commonly call it the tubulin dimer, to be extra clear about what we’re referring to, but the rest of the world does not necessarily use this term. Thus, it is important that you consider the context when “tubulin” is mentioned.
Since the tubulin dimers are all lined up in the same orientation, a different side is exposed at each end. At the end called the plus end in Figure 06-09, it is the beta-subunit that is exposed, whereas the alpha-subunit is exposed at the minus end. This is an important observation, as it tells us that there are going to be structural differences between the different ends. (This is unlike intermediate filaments, whose antiparallel subunit arrangement means that there is no obvious way to differentiate one end from the other structurally.) The structural difference between the ends of the microtubule is a key characteristic and influences microtubule function. This structural characteristic is also why we say that microtubules have polarity. This simply means the ends are different from each other. Labeling them as the plus and minus ends is not related to electrochemical charge in any way. Instead, this nomenclature is related to their propensity for growth. The plus end grows more easily, and faster, than the minus end does. This is the direct result of the subunits that are exposed at each end.
When researchers examined how the assembly of the polymer worked, they found that much like polypeptides and nucleic acids, microtubules grow by addition of new subunits at the ends of the polymer only. The tubular structure of a microtubule is assembled in a manner that can be quite striking. Assembly is shown in Video 06-02.
Microtubules and Dynamic instability
Microtubules are in a constant state of flux. They will grow, pause, and then shrink again in the span of a few seconds. They may shrink down to nothing or pause and then start growing again. Because of this constant state of flux, which shows that microtubules are dynamic (i.e., constantly changing from growth to shrinkage and vice versa), and because they are clearly very unstable (since they fall apart a lot), we say that microtubules undergo dynamic instability.
The discovery of microtubules dates back almost as far as the invention of the microscope, with observations of cilia and flagella in the late 1600s. However, it wasn’t until the 1980s that scientists developed the theory explaining dynamic instability.
Dynamic Instability in Vitro (i.e., in a Test Tube)
The simplest way to understand microtubule polymerization is to remove tubulin from cells and see how it works on its own in a test tube. In essence, this is what Mitchison and Kirschner did in 1984 when they first described dynamic instability. They discovered a few key features, which were then built on by other scientists as time went on. Here, we outline the basic theory of dynamic instability. Later, we will see how dynamic instability is central to the function of both actin and microtubules.
Tubulin dimers have a certain affinity for each other, and if there is a high enough concentration of tubulin in the test tube, they will start to polymerize into a polymer spontaneously. The polymer forms using intermolecular forces exclusively (i.e., no covalent bonding! See the introduction if you need a refresher on what this means).
This follows a predictable pattern and, on a graph, looks very similar to other chemical reactions (Figure 06-10). In essence, once you add enough tubulin to a test tube, the reaction starts to take place. At first the reaction is slow (lag phase), followed by an exponential phase of fast polymer growth. Like all reactions in a test tube, eventually this will reach equilibrium, and the concentration of tubulin that is incorporated into microtubules will stabilize and remain constant. Even so, there will still be dynamic instability going on in the test tube, as we know that in chemistry, at equilibrium, both the forward reaction and the reverse reaction happen at the same rate.
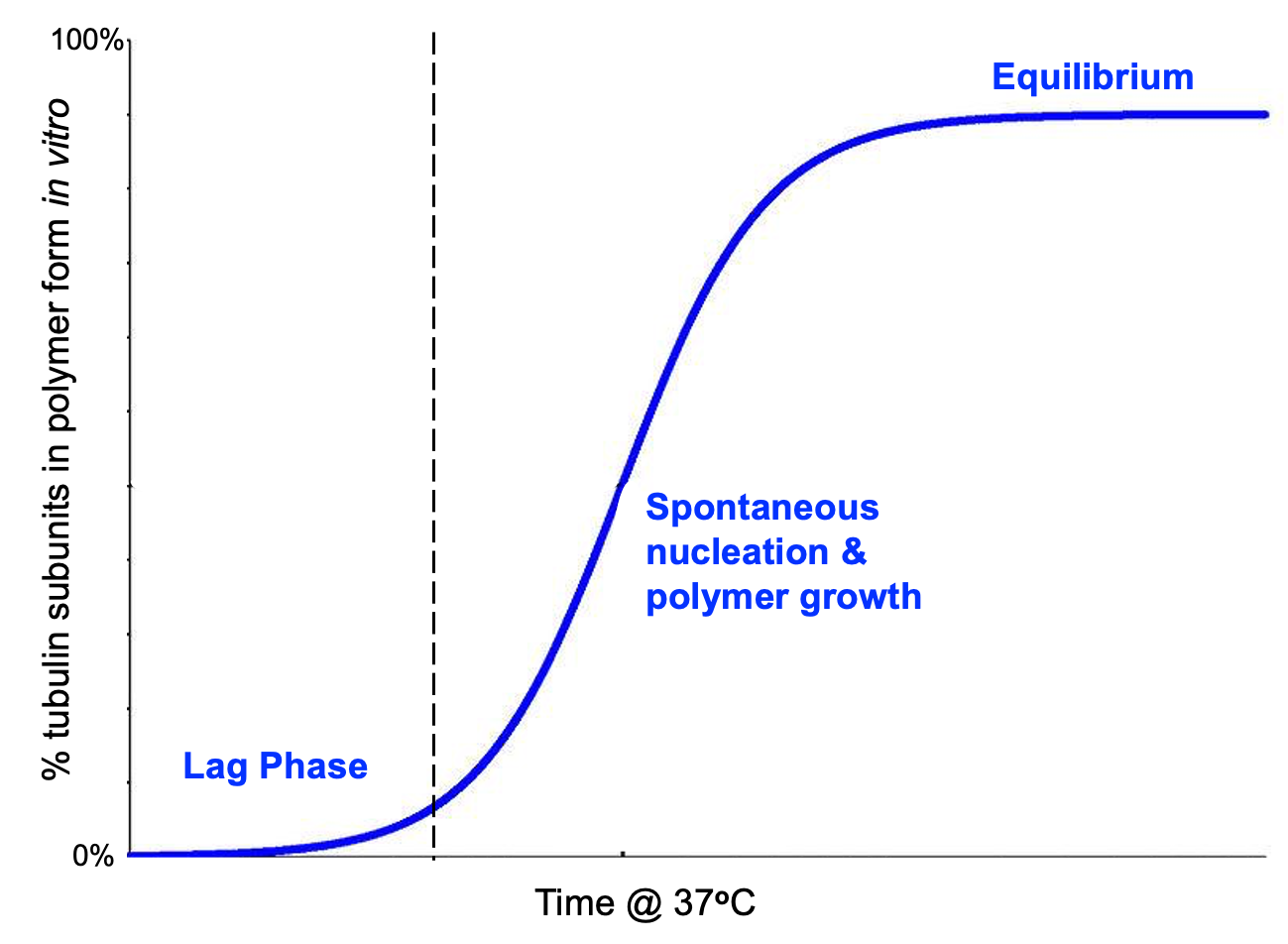
The lag phase is the slowest stage of the reaction in Figure 06-10. Initial nucleation is dependent on molecules running into each other in the right orientation, via random motion, in the aqueous solution of the test tube. Because of this, it’s easy to imagine that the concentration will have a strong effect on how this reaction proceeds. If the concentration of tubulin is too low, then it will be difficult for the tubulin subunits to find each other in solution to start the process. In the experiment that Figure 06-10 is based on, the concentration of tubulin is quite high, almost 20 times the concentration in an actual cell, and yet the lag phase is still quite slow. The lag phase is the rate-limiting step of the overall reaction.
If the concentration of tubulin is too low in the test tube, the process will not even start. The entire reaction essentially gets trapped in lag phase, and polymerization will not occur. Because of this, we say that there is a critical concentration (Cc) of tubulin required for polymerization to proceed.
If we examine the relationship between rate of assembly and concentration more closely, there is more to learn. First of all, we must remember that each microtubule has two ends, the plus end and the minus end, which have different structural properties. At the start of this section, we said those structural differences caused the ends to be different in terms of rate and likelihood of polymerization. We can visualize this when comparing the rate of growth to the concentration of tubulin we add to the test tube, as shown in Figure 06-11. The graph teaches us two things:
- Above the critical concentration, we see that the rate of assembly increases with increasing concentration of tubulin subunits in solution.
- There is a difference in both the critical concentration and the rate of assembly at the plus and minus ends.
This means that the plus end starts growing more easily than the minus end and also grows at a faster rate. Thus, Figure 06-11 provides the evidence for what we said earlier about the plus end being “more prone to growth” than the minus end. Note that in a test tube, where the minus end is unbound, the minus end is also able to grow, but it requires a higher concentration to get it started, and once it starts, it grows more slowly.
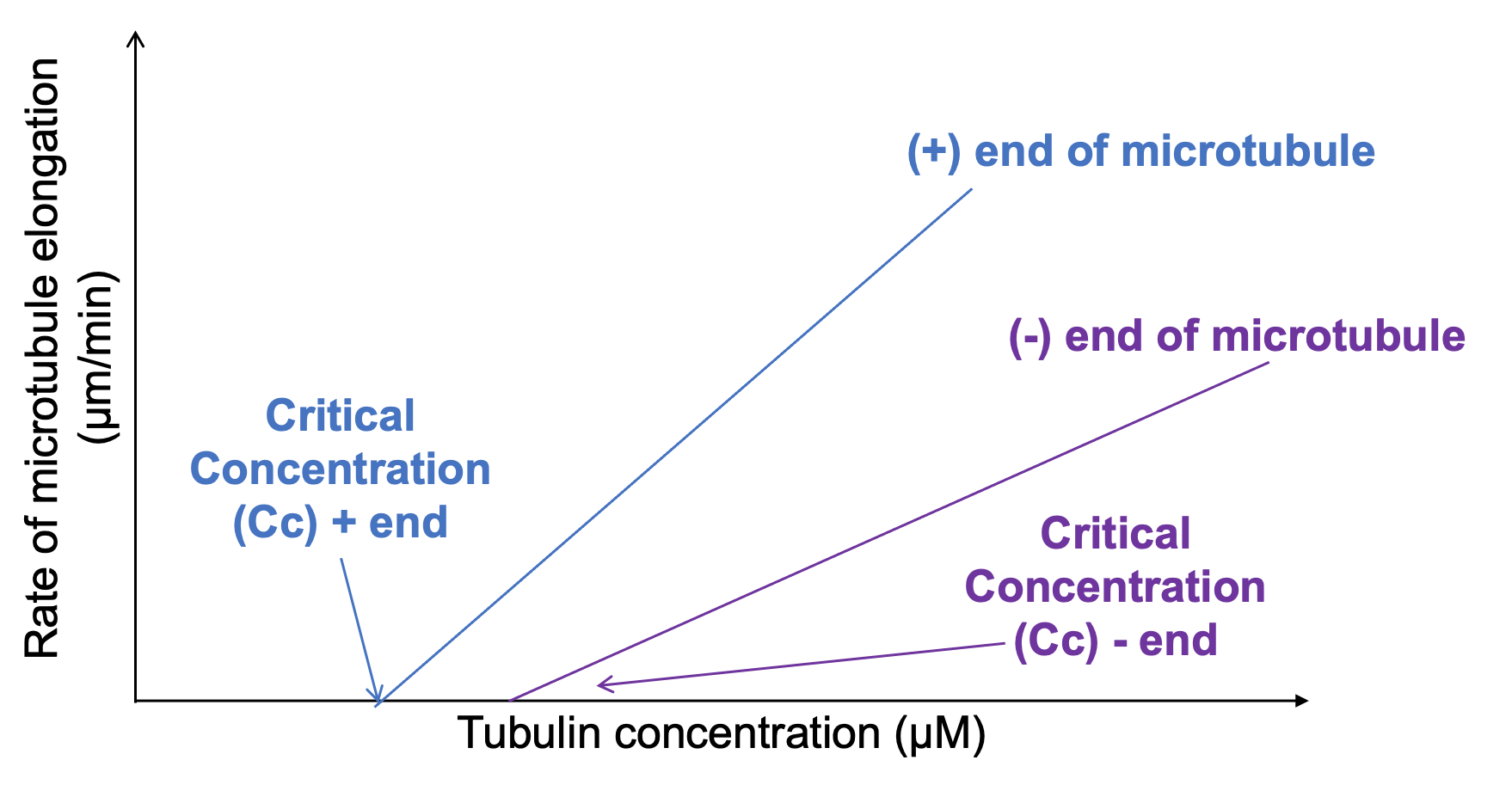
Interestingly, data collected on microtubule shrinkage rates show that unlike growth, the rate of shrinkage is not dependent on concentration. Shrinkage rate appears to be more or less constant at both ends at all concentrations. This is somewhat analogous to the fact that the rate at which a tower gets built is dependent on how many workers there are to do the work. However, destroying a tower has very little to do with the number of workers…once the primary supports are gone, the rest will come down very quickly.
Dynamic Instability in Vivo (i.e., inside Cells)
Now that we understand dynamic instability in the simpler environment of the test tube, we need to consider it in the context of the cell. The cell, of course, is a much more complex place, and the polymerization of microtubules will be heavily influenced by their environment. Local tubulin concentrations may go up and down as nearby microtubules grow and shrink. Additionally, accessory proteins will bind to both the tubulin dimers and the polymers to influence overall microtubule behavior based on the needs of the cell.
One important aspect of tubulin dynamics that we have not yet discussed is the difference between tubulin dimers that have GTP bound to their beta-subunit and those that have hydrolyzed their GTP into GDP. (Remember that the alpha-subunit can neither release nor hydrolyze its GTP, so we can ignore it here.) The hydrolyzation of GTP in tubulin causes a change in the conformation of the protein, which impacts its function. Here’s what you need to know:
- While both forms of tubulin are able to polymerize, the GTP-bound tubulin dimers are considered to be the activated form. GTP-bound tubulin has a higher affinity for the microtubule polymer, which results in a lower critical concentration, and it assembles at a faster rate compared to GDP-bound tubulin (which is often called the inactive form, even though that’s not entirely accurate).
- GTP tends to be in excess concentration in the cytosol. As a result, GDP-bound tubulin that is not bound up in microtubules is immediately reactivated by replacing the GDP in the beta-subunit with GTP. So the concentration of free-floating, GDP-bound tubulin tends to be quite low in the cell.
- As a result, in the cell, we really only need to consider GTP-bound tubulin during microtubule assembly, but both GDP- and GTP-bound tubulin play a role at other points in the life cycle of the microtubule.
- As soon as a tubulin dimer binds and becomes part of a microtubule, the beta-subunit begins to slowly hydrolyze its GTP (Figure 06-12). The hydrolysis of GTP to form GDP reduces the affinity of the binding of the tubulin dimers to each other within the microtubule. This means that over time, the affinity of the tubulin dimers for each other (and, as such, the bonding energy holding the polymer together) will go down.
- When hydrolysis of GTP occurs more slowly than the new monomers can be added at the plus end, the microtubule will grow at a maximum rate (i.e., it will be in elongation phase). As a result, there will be a buildup of GTP-bound beta-subunit at the plus end of the microtubule. This accumulation is known as a GTP cap at the growing end.
- The lower affinity of GDP-bound tubulin dimers for the microtubule means that if growth slows down to the point that there are GDP-bound tubulin subunits exposed at an end of the polymer, the entire microtubule is more readily disassembled. The lower affinity of the GDP-bound monomers is due to a slight conformation change in the dimer, causing the protofilament to bend slightly (Figure 06-12 and Video 06-03).
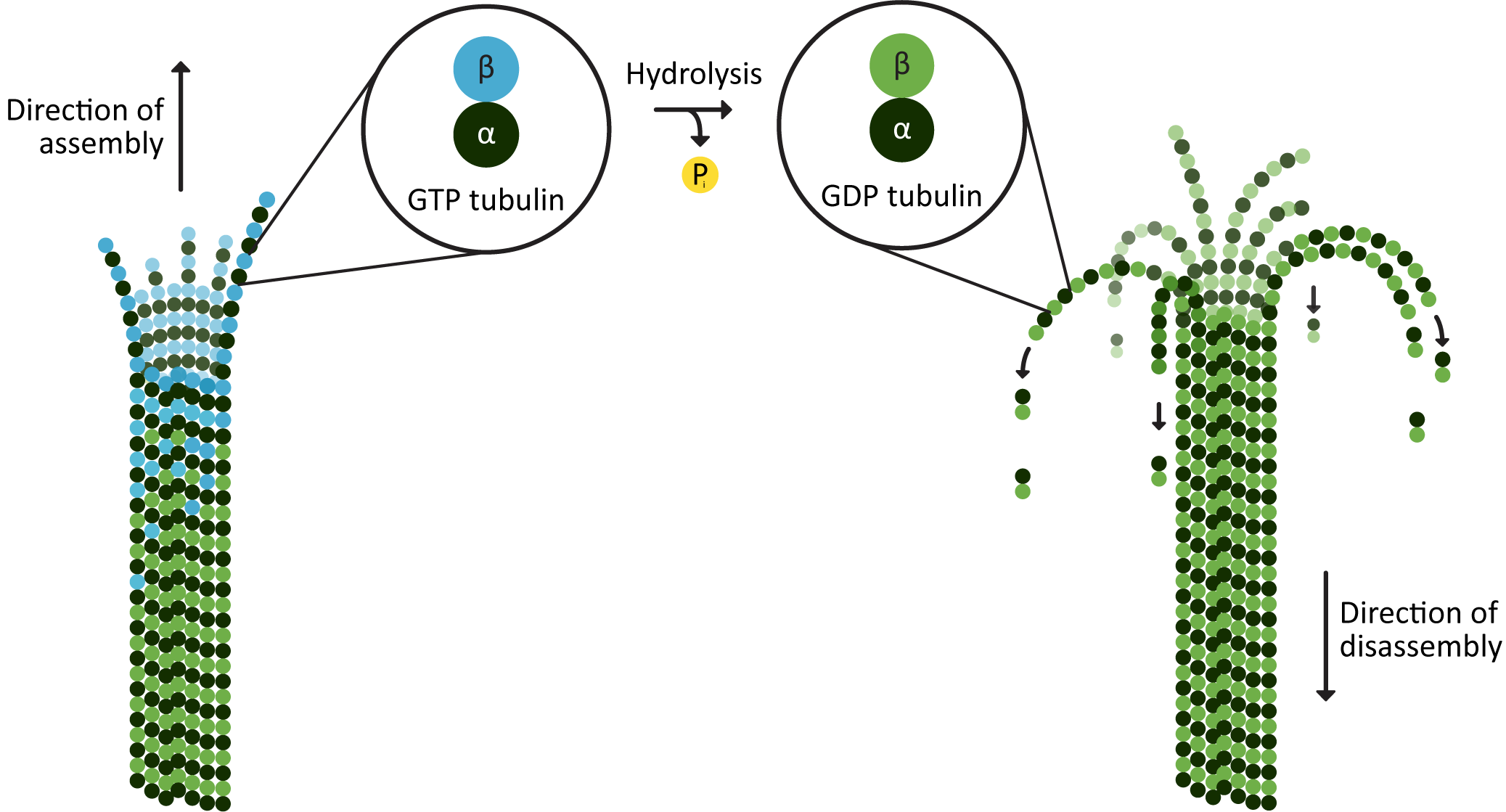
The loss of the GTP cap usually results in rapid shrinkage of the microtubule (Video 06-03). However, this may not be the end of it. If there is a high enough local concentration of activated tubulin, the microtubule can be rescued and then start to grow again (Figure 06-12). This means that if we watch microtubules over time, they are constantly changing length. This is the basis for the idea of dynamic instability, and it can be observed in fluorescence microscopy (Video 06-04).
Microtubule Function
Microtubules have a wide variety of functions in the cell. Their most prominent ones include the following:
- acting as the “highways” on which vesicles and other cargo are transported,
- helping control and maintain specific cellular shapes,
- providing sites to anchor complexes and proteins in specific regions of a membrane,
- helping maintain organelle shape and location,
- coordinating the division of the DNA during mitosis, and
- forming the structural basis for eukaryotic cilia and flagella.
Each of these functions will require precise control of the microtubules so that they are always in the right place, at the right time, doing the right thing for the circumstances. However, if growth and shrinkage are dependent on conditions and protein chemistry, then how is the cell able to control this? The answer is that the cell controls the conditions in order to promote growth, maintenance, or shrinkage as required. In this section, we discuss the most prominent ways that this is done by the cell.
Microtubule Organizing Centers (MTOCs) Promote Growth
In many cells, especially animal cells, microtubules are observed growing from a specific site, at or near the center of the cell. This is known as a microtubule organizing center (MTOC) (Figure 06-13). In animal cells, it is also sometimes called the centrosome. MTOCs are sites where microtubule growth is specifically promoted through the use of a third type of tubulin, known as gamma-tubulin.
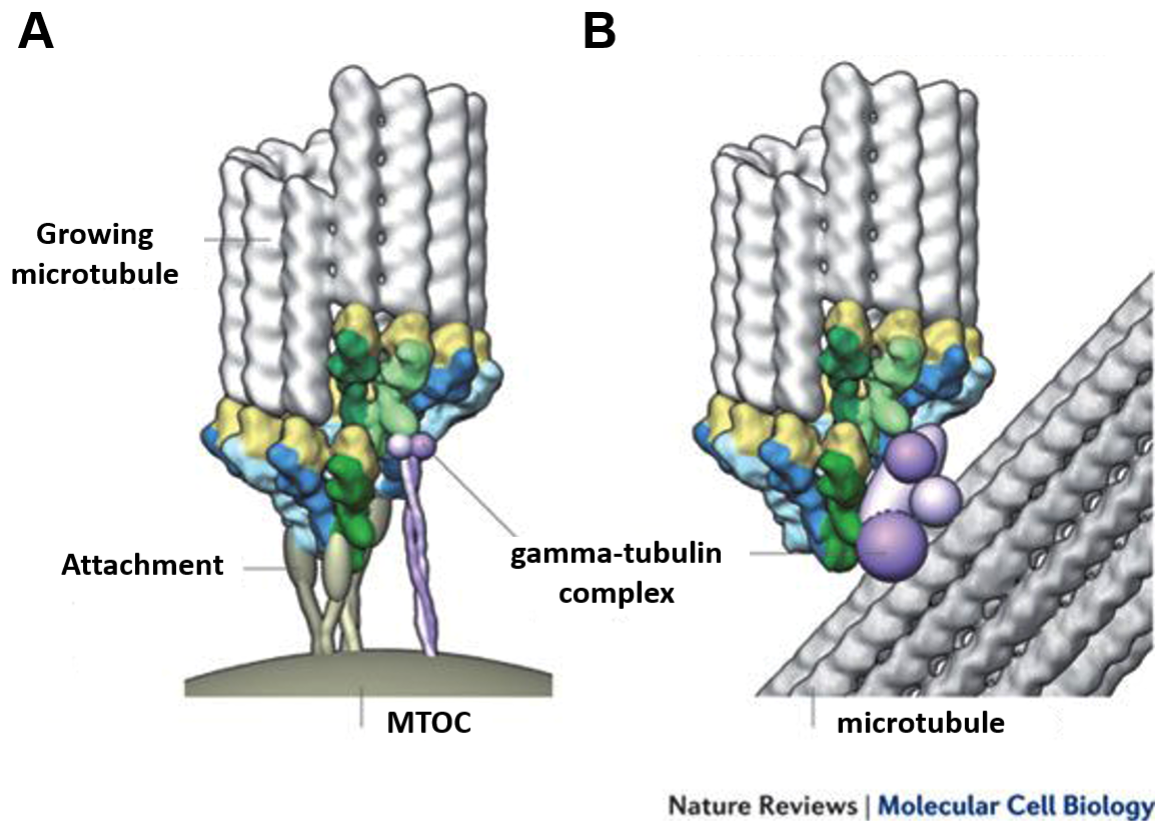
Gamma-tubulin helps promote microtubule growth by providing a premade template for the tubulin subunits to bind to. Having this template reduces the “lag phase” of the growth curve from Figure 06-10, which is the slowest stage of microtubule formation. An additional result is that the critical concentration required to promote assembly is lowered at these nucleation sites. As such, microtubules will grow more easily at these sites than they will elsewhere in the cell. In this way, the gamma-tubulin complexes help the cell control not only when but where microtubules grow.
A side effect of this method for promoting microtubule growth, which is evident in Figure 06-13, is that the minus end of the microtubule is bound to the gamma-tubulin ring. As a result, in most cells, the minus end is not free for growth or shrinkage, and it is only the plus end that is used for dynamic instability. However, there are important exceptions to this rule, which will be highlighted in Chapter 8.
Figure 06-13 also highlights that there are two ways that the MTOC can be arranged in cells: centralized (as we see in most animal cells, some fungi, and during mitosis in virtually all cells; Figure 06-13A) or decentralized (as we see in plant cells and some fungi in interphase; Figure 06-13B). Let’s talk about each of these a little bit more.
Centralized MTOC
Many cell types use centralized MTOCs in interphase, while others do not. In mammalian cells, which have a centralized MTOC throughout their life cycle, we call it the centrosome. The centrosome usually includes a pair of centrioles and their associated proteins (Figure 06-14A). Centrioles are also made of tubulin dimers, and their role is somewhat murky in a lot of cases.
During mitosis, all eukaryotic cells have two centralized MTOCs, often called the spindle poles, which coordinate the proper placement of microtubules for the separation of the chromosomes (Figure 06-14B).
Eukaryotic cells with cilia and/or flagella will have additional MTOC called basal bodies. These are a pair of centrioles found at the base of each cilium or flagellum that promote the growth of the microtubules within the structure (Figure 06-14C). Cilia and flagella are fascinating structures that are also more dynamic than we give them credit for. For example, they are often resorbed before mitosis and then regrown afterward.
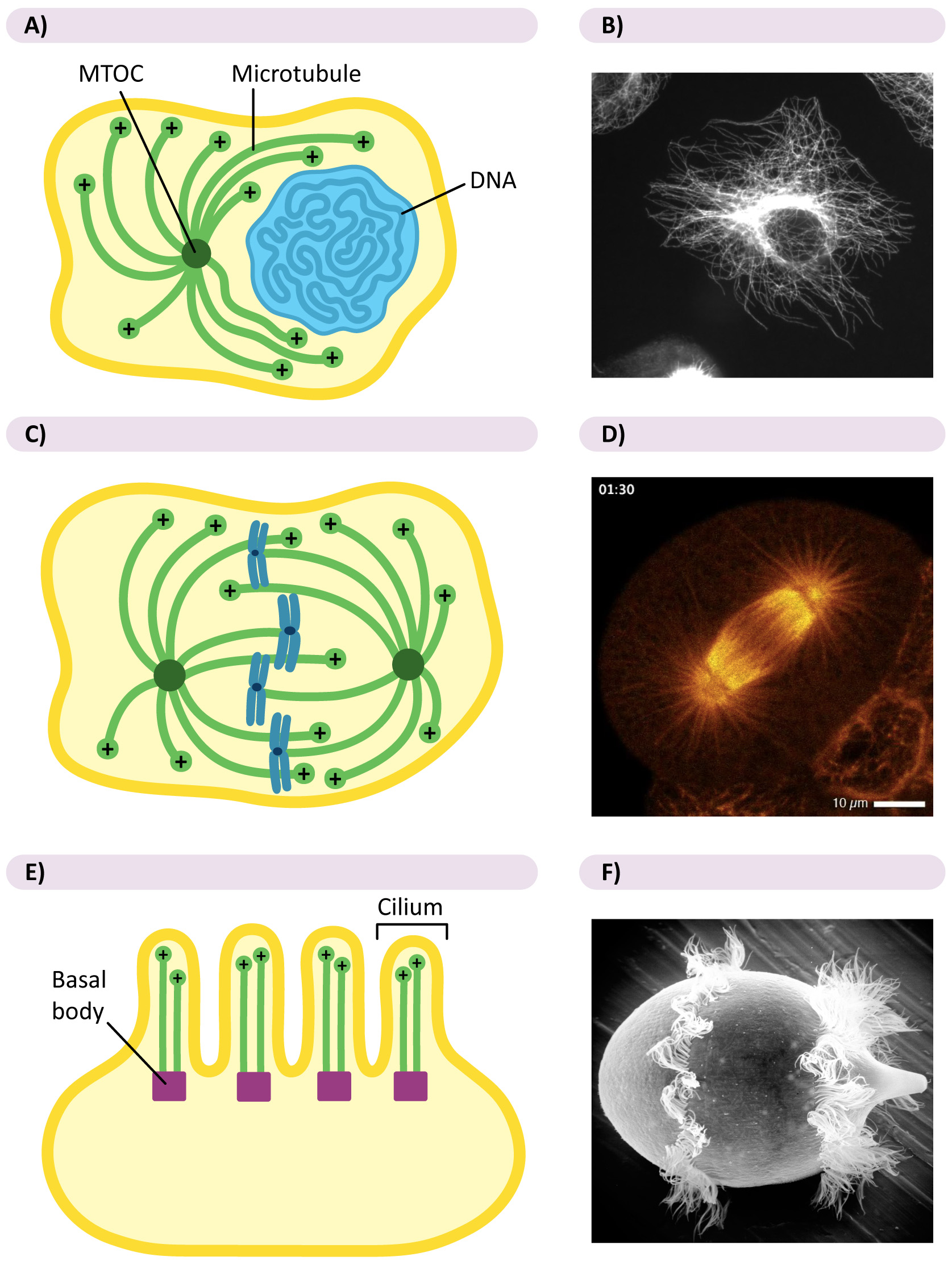
Decentralized MTOCs
- In plants and fungi, which do not have a centralized MTOC in interphase, the gamma-tubulin rings are generally carried along preexisting microtubules via motor proteins. With MTOCs present throughout the cell, microtubule growth is promoted evenly throughout the cell. The arrangement of microtubules in Video 06-04, above, is the product of decentralized MTOCs. It is a plant cell in interphase.
- During mitosis, cells that normally have decentralized microtubule growth will collect all of their gamma-tubulin into two MTOCs, which then act as spindle poles for the mitotic spindle so that cell division can take place.
Caps and Other Proteins That Control Microtubules
In order to harness the power of the microtubules and their capacity for dynamic instability, the cell uses a variety of proteins to control them. Some examples include the following:
- Nucleating proteins: Gamma-tubulin helps promote the growth of new microtubules.
- Stabilizing proteins: XMAP215 helps stabilize preexisting microtubules and allows them to grow more efficiently.
- Severing proteins: Katanin is a protein that severs microtubules somewhere in the middle of the filament.
- Destabilizing proteins: Kinesin 13 binds at the plus end and promotes disassembly.
- Monomer sequestration: Stathmin binds to unpolymerized tubulin dimers, which inhibits microtubule growth.
- MT organizing proteins: A variety of proteins, such as Tau and MAP2, are involved in building and stabilizing specific arrangements of microtubules known as arrays.
- Connecting proteins: Plectin binds to both microtubules and intermediate filaments, thus connecting the different cytoskeletal networks.
- Protein caps: Used to stabilize microtubules to stop them from growing and shrinking.
- Motor proteins: Used to help vesicles travel down the microtubule, among other specialized roles.
We aren’t going to discuss all of these, as there simply isn’t enough time. We will highlight only two: protein caps in this section and motor proteins in the next section.
Protein Caps
If a microtubule is required for a long period of time in the same place, it must be stabilized to reduce or eliminate dynamic instability. We’ve already seen an example of how this can be done at the minus end—the gamma-tubulin is acting as a “cap” that stops the microtubule from being able to depolymerize from that end. A protein cap can also be added to the microtubule at the plus end so that the microtubules cannot depolymerize from there either. Microtubules that are capped at both ends are generally quite stable and remain in place for as long as the cells need them.
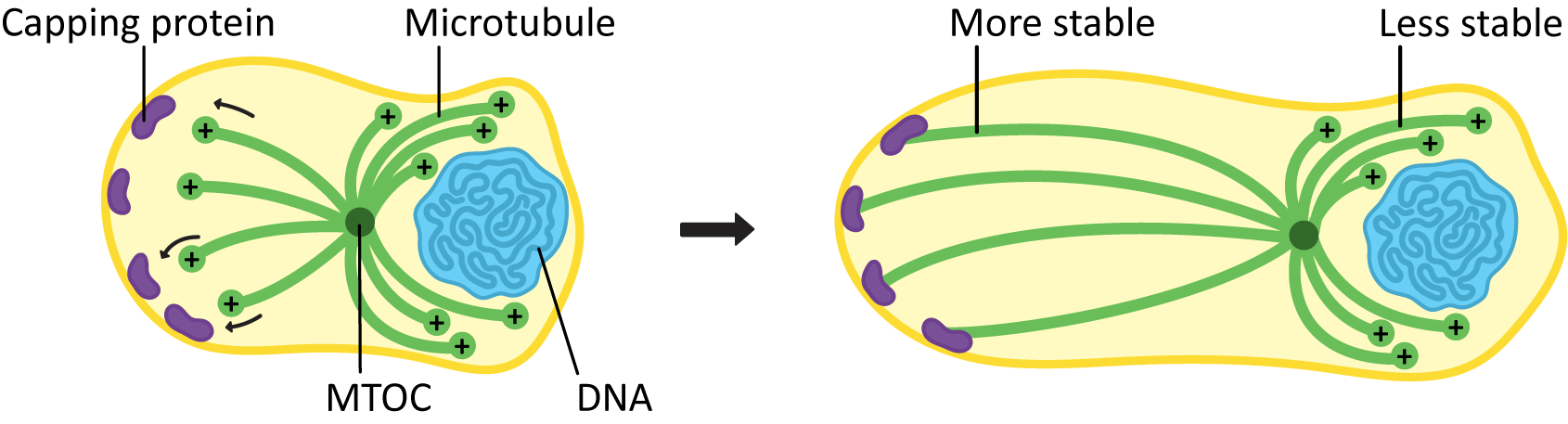
Stabilization of microtubules can be an important part of cellular development. Microtubules bind to capping proteins at the cell surface to influence the final shape and structure of a cell, as seen in Figure 06-15.
In cells like axons, which have long projections that must be maintained, the stabilization of microtubules in the axon (in addition to the production and assembly of neurofilaments) is an important part of how the axons develop. Loss of the microtubules will have the same effect as loss of the neurofilaments in that the axon may start to retract. This will cause the cell to lose its connections to the rest of the neural network and die.
In addition to their role in stabilizing the axon, the microtubules in these long axons are required to transport vesicles and other cargo from the cell body to the plasma membrane at the axon terminal (Figure 06-16). Motor proteins are used for this, which we will describe next.
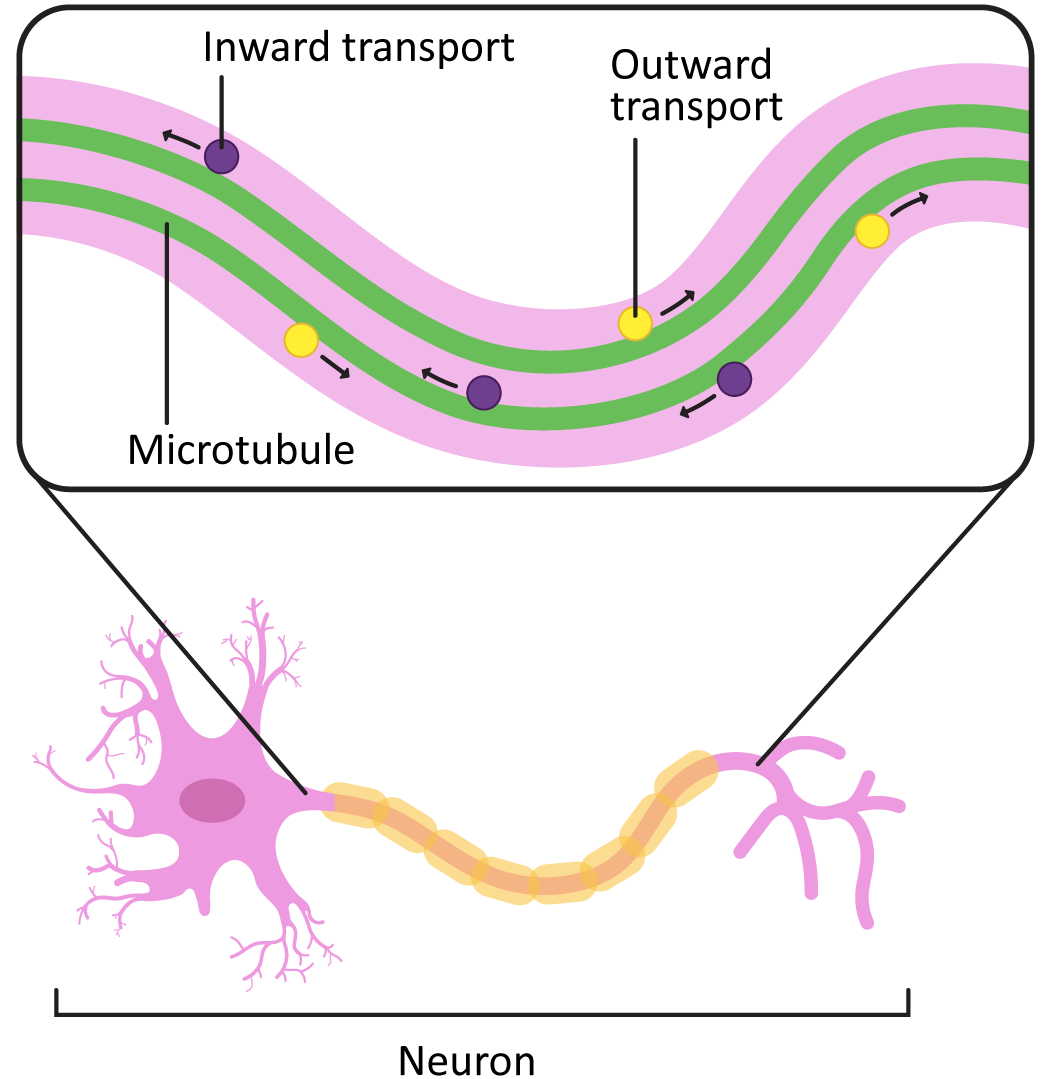
Motor Proteins
The ability to move directionally along microtubules is a key requirement for many cellular functions, including cargo trafficking, organellar positioning, and mitosis. This is where the polarity of the microtubules becomes critical. Microtubule motor proteins have the ability to bind to the microtubule and move along it in a specific direction (using energy provided by ATP).
There are two classes of motor proteins that use microtubules to move (Figure 06-17):
- Kinesins—most commonly move toward the plus-end of microtubules (see Video 06-05)
- Dyneins—move toward the minus-end of microtubules
Since microtubules are polar molecules, due to the linear arrangement of the tubulin dimer, the motor protein can “walk” along the microtubule “highway,” carrying its cargo toward the plus end (in the case of kinesins) or toward the minus end (in the case of dyneins).
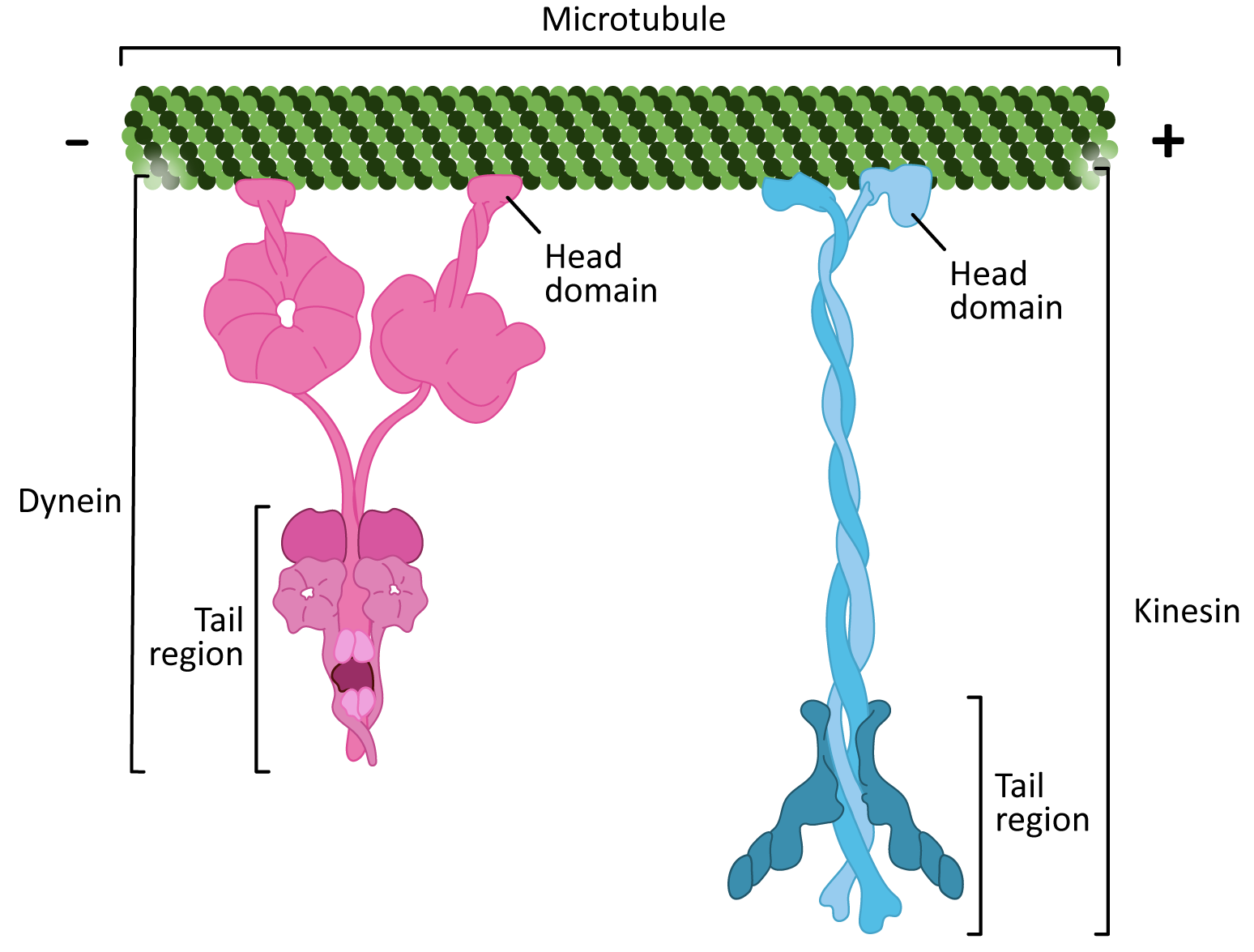
There are some interesting similarities between the two classes of microtubule motor proteins (Figure 06-17). Kinesins and dyneins are both multisubunit complexes. They have globular head groups that attach to the microtubule. They also have a tail region that attaches to cargo. Both classes of motors are powered by ATP and have some kind of “stepping” cycle linked to ATP hydrolysis.
However, as you can see from Figure 06-17, their shape is very different. Their mechanism of action is also quite different. Kinesin “walks” along the microtubule, moving one motor head and then the other (Video 06-06). On the other hand, dynein appears to use more of a “strut”-like motion (Video 06-07).
Motor proteins transport a number of different types of materials, including the following:
- vesicles
- proteins and/or protein complexes
- protein-mRNA complexes (also known as RNP particles)
- membrane-bound vesicles and organelles (including membranes of the ER and Golgi)
- other microtubules, as in flagella, cilia, and other motile microtubule arrays
Motor Proteins and Organelle Positioning/Maintenance
One question that is usually overlooked when studying the organelles of the cell is “How did they get to be shaped like that?” Membranes are not going to spontaneously form the flattened pancake of the Golgi apparatus or the interconnected tubular structure of the ER. So how does that structure happen?
Another question we rarely consider is how the different organelles manage to maintain their position in the cell. They are subject to gravity, like everything else on the planet, so why aren’t the nucleus, mitochondria, and the rest of the organelles sitting on the bottom?
The answer to both of these questions is the same: molecular motors. The molecular motors have an important role to play in both the maintenance of structure and the location of the organelle within the cell.
Organelles have several motors attached to them. They likely have both dynein and kinesin, as well as the actin motor, myosin. These will work together to properly position all of the parts of the organelle in the 3D space of the cytosol. Let’s consider two membrane-bound organelles:
- The Golgi apparatus maintains a position near the nucleus in mammalian cells. This is also near the interphase centrosome. Dyneins have been shown to play an important role in the maintenance of this position. When microtubules are experimentally depolymerized, the Golgi has been observed to fragment and float away from its original position.
- On the other hand, the endoplasmic reticulum, which extends throughout the cytoplasm in most cells, has a different reaction when microtubules (MTs) are experimentally depolymerized. In this case, the ER has been shown to become more spherical and retract toward the nucleus. The ER tubules are moved outward by plus-end kinesin motors, walking toward the plus end of microtubules.
Topic 6.3: Actin Filaments
Learning Goals
- Compare and contrast dynamic instability in actin and microtubules.
- Correlate in vivo microfilament polymerization and organization at the cell cortex with its function of deforming the plasma membrane and regulating cell shape.
- Relate how actin-binding proteins influence actin filament polymerization and organization to regulate filament function (e.g., cell motility).
- Interpret results from experiments using drugs that disrupt microfilaments and compare with equivalent experiments that disrupt microtubules.
- Explain the function of myosin motors and their role in contractile bundles as well as in other cellular contexts, such as cytoplasmic streaming or muscle contraction.
The final cytoskeletal element that must be discussed is actin filaments (also known as microfilaments, filamentous actin, f-actin, or just plain “actin”). In animal cells, these filaments are major components of the peripheral cytoskeleton, the cell cortex, and complex contractile systems such as muscle.
Actin filaments can form either stable or dynamic structures, depending on how they are cross-linked to other proteins. As a result, the array of actin-binding proteins (ABPs) that exist are considered extremely important to understanding actin function.
Actin Structure and Filament Formation
Like microtubules, actin filaments are a polymer of actin subunits (sometimes called actin, actin monomers, globular actin, or g-actin). The subunits line up end to end and polymerize in such a way that different sides of the subunit are exposed at either end (Figure 06-18). This means that the filament has a directionality to it, so actin filaments are also referred to as polar molecules (once again, this has nothing to do with charge or other chemical properties per se).
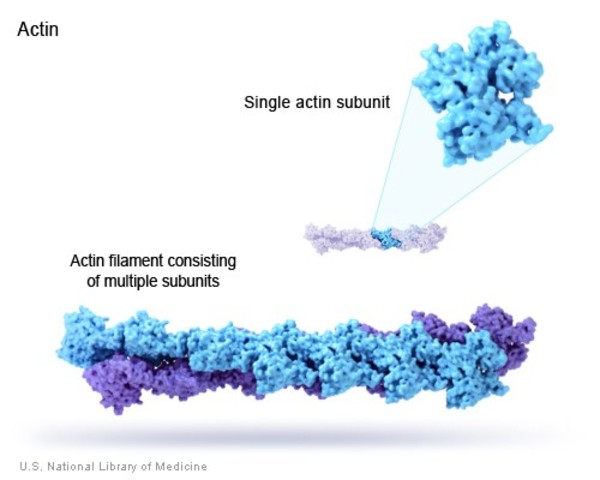
Like microtubules, actin also is capable of dynamic instability even though actin researchers do not commonly discuss actin in those terms. This is, in part, due to the large group of ABPs that are used to control actin monomers and filaments. As a result, the functions of actin filaments and microtubules are very different, as you will see throughout this topic.
Actin and Dynamic Instability
It’s important to note here that in vitro (i.e., in a test tube) actin and tubulin behave very similarly. This means the following:
- They both undergo dynamic instability.
- The plus end has a higher affinity for monomers than the minus end, resulting in
- a lower critical concentration and
- an increased rate of assembly once polymerization starts.
Like tubulin, actin uses chemical energy to drive the process of polymerization (Figure 06-19). However, in this case, ATP is used instead of GTP. In a living cell (i.e., in vivo) there is a high concentration of both ATP and GTP in the cytosol. Thus, it is relatively straightforward to keep the actin monomers in their “active,” ATP-bound state, which favors polymerization. Video 06-08 shows a molecular model of the polymerization of actin.
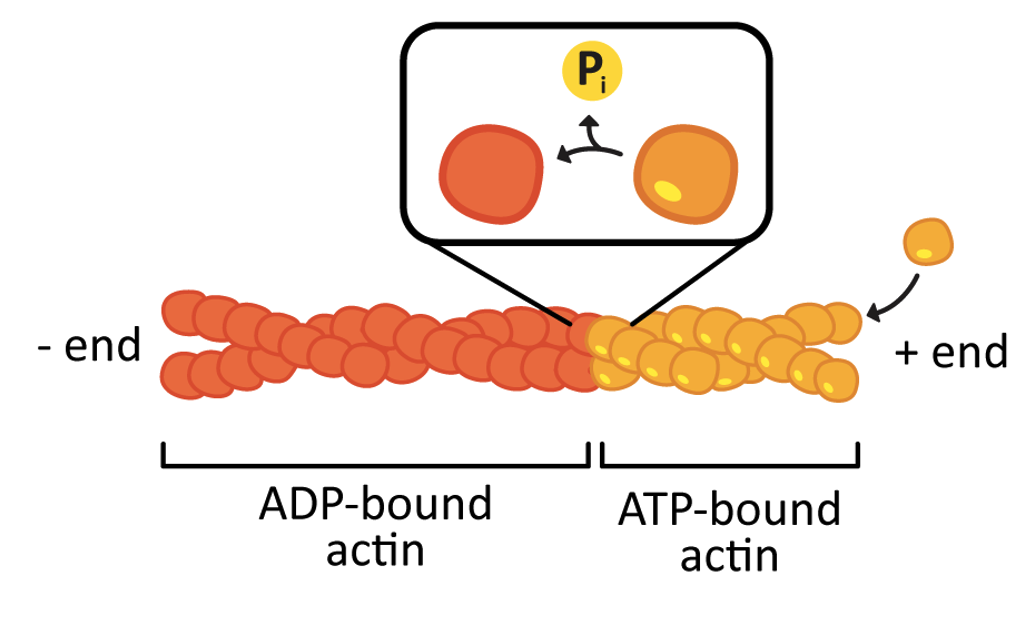
Actin Function
Actin-Binding Proteins (ABPs)
The cell uses many of the same strategies to control actin filament formation, as we saw in the section on microtubules. Proteins known as ABPs are used extensively to control where and when actin filaments form in the cell. In fact, far more proteins exist to control actin than have been found to control microtubules. This is due, in part, to the number of highly specialized roles that actin plays in different cells. Of course, actin filaments have different functions in the cell compared to microtubules, so the types of binding proteins and the structures formed by actin filaments are distinct from those of microtubules.
Similar to microtubules, actin formation is modulated by the following:
- Controlling where an actin filament forms: Decentralized nucleation sites provide locations to polymerize actin filaments easily. Unlike microtubules, there are no examples of a centralized organizing center for actin. In actin, there are two major types of actin nucleation sites:
- Branching networks—formed when Arp2/3 binds to the side of a preexisting actin filament. We will see this when we discuss cellular locomotion.
- Parallel networks—formed when formin and profilin bind to actin.
- Stabilizing actin filaments using protein caps: Many of the structures formed by actin must be maintained for the long term. ABPs bind to the end of the filament and act as caps to prevent disassembly (Figure 06-20). Examples of these long-lasting, stable actin structures include the microvilli, found in the intestinal tract, and the actin filaments that form the basis for muscle contraction (along with the actin motor protein, myosin).
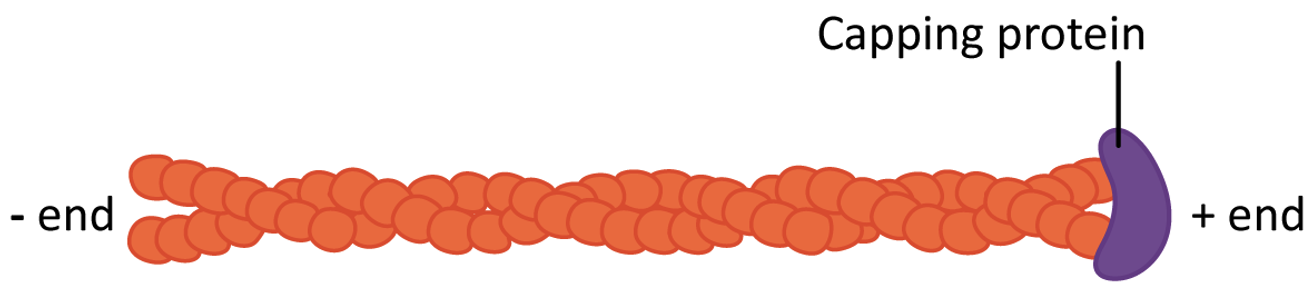
By controlling where actin forms and when/where it gets stabilized, ABPs and actin can form several different types of actin networks in the cell (known as arrays; Figure 06-21):
- Parallel bundles—filaments are closely spaced and have the same polarity (directionality). For example, parallel bundles are found in filopodia or microvilli. The proteins formin and profilin build these arrays with additional proteins to cross-link.
- Contractile bundles—filaments are arranged antiparallel and are cross-linked by stabilizing proteins. Molecular motors drive the “contraction” of these arrays. Examples include stress fibers in cells and in the contractile ring that splits apart daughter cells during cytokinesis.
- Cross-linked gel—random orientation of fibers linked at the crossing by filamin. Actin gel is a major component of the cell cortex of most cell types and plays a big role in locomotion. Arp2/3 is used to build the filaments in these arrays, and additional proteins are used to cross-link and stabilize.
In association with these internal arrays, actin has also been observed to attach to a variety of plasma membrane proteins, which allows it to help shape the cell and to respond to the cellular environment. Actin is thought to play a key role in cell shape for all cells, but in animal cells, these arrays are the primary driver for most cell shapes.
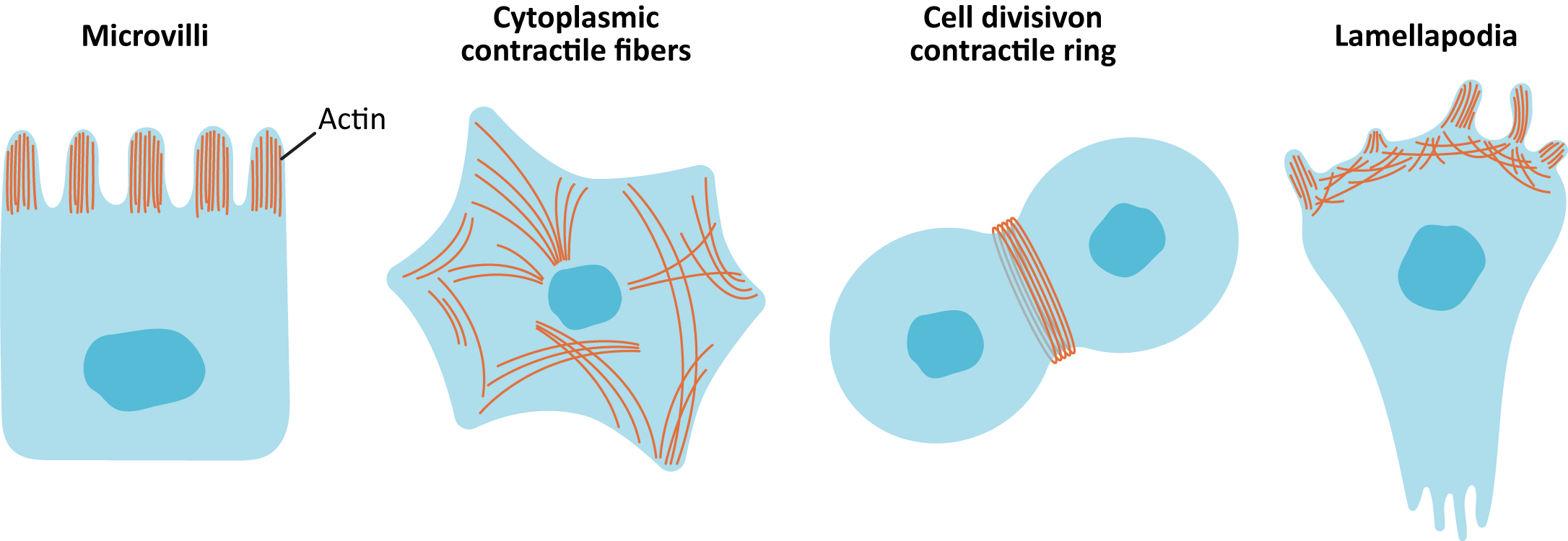
Myosin Motor Proteins
Just like microtubules, actin filaments also use motor proteins to move cargo along the actin filament. Myosins are a large and diverse class of motor proteins that use actin filaments as their substrate.
Myosin motors are involved in a number of cellular functions (Figure 06-22), including the following:
- vesicle transport (Myosin I is the most important myosin for this, but others also get involved.)
- organelle positioning
- cytokinesis
- cellular locomotion and shape
- phagocytosis
- muscle contraction (Myosin II: We know quite a lot more about this myosin than any of the others, as quite a lot of research is done on muscle function.)
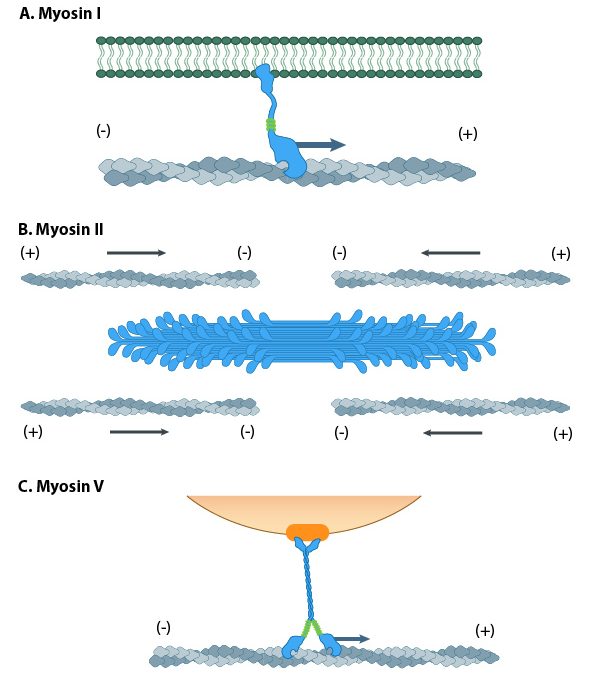
The myosin family of proteins is very large. There are as many as 18 different types of myosin motors found in eukaryotes. Most of these myosins move toward the plus-end of actin filaments; however, there are some exceptions. One subtype, called myosin IV, has been shown to move toward the minus-end. Myosin motors are complexes of several polypeptide chains (Figure 06-22). Some subtypes have one motor head, and some have two. However, the motor head functions the same way regardless of how many there are.
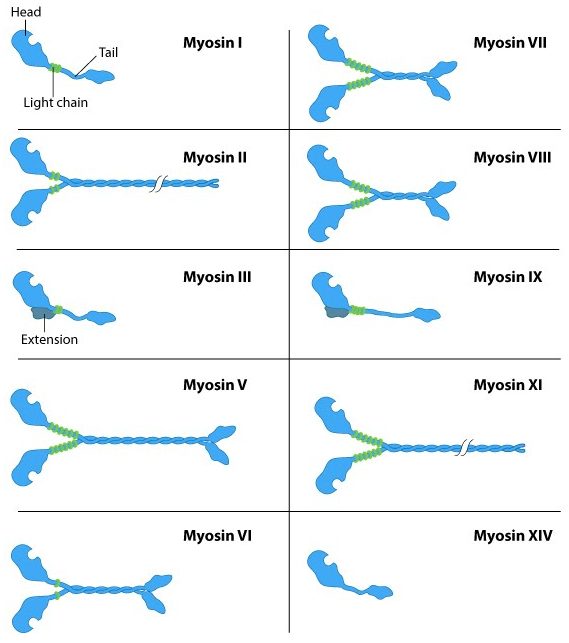
In a nutshell, they bind to the subunits of the actin filament, pull their motor head in, and then release with the help of ATP. Different myosins appear to move slightly differently, so it’s more difficult to find a single video to represent them all. Muscle myosins (myosin II) work through a cycle that includes a “power stroke” that pulls the actin filament in toward the center of the muscle (Video 06-09). Myosin V, on the other hand, appears to use a very different mechanism that is similar to the movement of a Slinky toy (Video 06-10).
Like the microtubule motors, myosin motors require ATP in order to function. The ATP hydrolysis helps prime the motor head to prepare for the next time it binds and pulls on the filament.
Interestingly, no ATP is required for binding to the actin filament, but every other step of the process requires either ATP or ADP. This is the opposite of the microtubule motors, which cannot bind to the microtubule (MT) unless they are bound to ATP.
As a result of this unique situation, myosin motors will freeze in place if the cell runs out of ATP, which happens at the point of death or shortly thereafter. Muscle contraction is dependent on successive rounds of binding and unbinding of myosin, so without ATP, the myosin binds and causes the muscles to become really rigid right after death. This is a well-known phenomenon called rigor mortis. It is commonly referenced in detective shows, especially those with a focus on forensic science.
Example: Cellular Locomotion
Locomotion in animal cells is a good example of how precise control of dynamic instability can be used to the cell’s advantage. Cellular locomotion is a complex interplay of holding on to the substrate, building a network in one area of the cell, and deconstructing the network in another area (Figure 06-24). Every step of this process requires ABPs to make sure that it all goes according to plan.
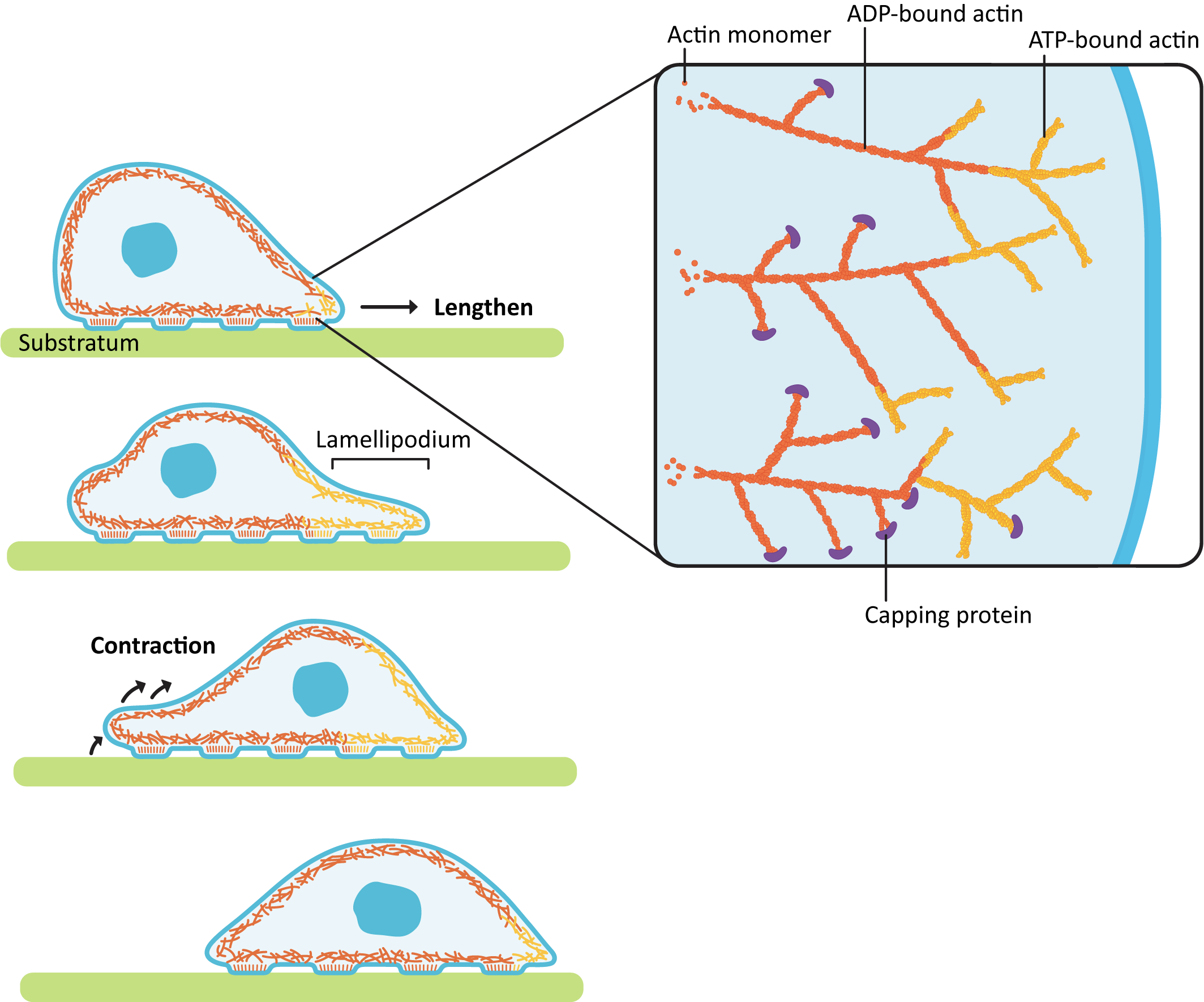
The act of forming the membrane protrusion at the leading edge requires actin polymerization directly underneath the plasma membrane. Nucleation of new actin filaments at the leading edge is mediated by Arp2/3 complexes. As you may recall, Arp2/3 binds to the side of a preexisting filament so that a branched array is formed (Figure 06-24). The growing actin network is what pushes the leading edge forward, creating membrane waves, or ruffles, called a lamellipodium (plural lamellipodia). At the same time, the polymerized actin at the back end of the leading edge (i.e., on the far side of the branched network from the membrane) is depolymerized so that free actin can be moved forward. This ensures that the cell continues to have access to free actin for polymerization at the leading edge.
As the leading edge is being pushed forward by the lamellipodia, the back of the cell also needs to be pulled forward, so the cell moves forward instead of just flattening out. This contraction is accomplished by a network of actin and myosin motors (sometimes referred to as the actomyosin network) that contract within the cell body, creating tension. As it does this, the proteins holding onto the substratum at the rear release so that the contraction can happen, and the cell moves forward.
Cellular locomotion is well illustrated by Video 06-11, filmed in the 1950s using a light microscope attached to a film camera. In it we see a white blood cell chasing, capturing, and engulfing a bacterium.
Studying Cells: Drugs That Influence Actin and Microtubule Function
Before we leave our discussion of the cytoskeleton, we must once again explore how scientists study the function of the cytoskeleton. As we’ve seen before, a commonly used tactic in biology is to disrupt a biological system and observe the results. You may not be surprised to know that both actin and microtubules are essential cellular structures. So mutational analysis is not a very good option for experimental study.
However, since the cytoskeleton is so important, especially as a key player in cell division and muscle contraction, it has become the target of many natural toxins produced by a variety of plants, animals, and fungi in order to protect themselves from other organisms in their environment. As scientists, we are able to exploit these compounds to our advantage and use them to temporarily disrupt the cytoskeleton and then observe the impact on cellular function. Table 06-01 shows the most commonly used compounds and how they impact the cytoskeleton when applied.
Chemical Compounds that Impact Microtubules or Actin Filaments | ||
---|---|---|
Microtubule-specific | ||
Compound | Action | Source |
Paclitaxel | Binds to microtubule and prevents disassembly | Bark of Pacific yew (Taxus brevifolia) |
Colchicine | Binds to unpolymerized tubulin and prevents assembly | Autumn crocus (Colchicum autumnale) |
Vinblastine | Binds to unpolymerized tubulin and prevents assembly | Madagascar periwinkle (Catharanthus roseus) |
Oryzalin | In plants only; binds to unpolymerized tubulin and prevents assembly | Synthetic herbicide in the dinitroaniline family |
Actin-specific | ||
Compound | Action | Source |
Phalloidin | Binds to actin filament and prevents disassembly | Death cap mushroom (Amanita phalloides) |
Latrunculin | Binds to unpolymerized actin and prevents assembly | Sea sponges in the Latrunculia and Negombata families |
Cytochalasin B or D | Caps the plus end of actin filaments | Fungal plant pathogen of the Helminthosporum genus |
What we hope you will notice in the table is how varied the effects of the drugs are. While some of them interact directly with the polymerized microtubule, others work by disrupting the chemical equilibrium through interactions with the monomer. This shows how essential dynamic instability is to the proper function of both actin and microtubules.
One final note: There is often confusion between the difference in “capping” and “stabilizing,” especially with respect to the function of these compounds. A capped filament is also stabilized, as the polymer cannot fall apart. But it is possible to stabilize without capping. A perfect illustration of this is the difference between paclitaxel, which stabilizes microtubules without capping so that they may still grow (but not shrink), and something like cytochalasin, which acts as a protein cap at the plus end of the actin filament. Cytochalasin stops the microtubule from growing or shrinking, whereas paclitaxel only inhibits shrinking but not growth.
Chapter Summary
By now, we hope you agree that the cytoskeleton is an intriguing cellular system that helps cells to take on very specific functions. If not for the cytoskeleton, cells would largely be shaped like amorphous blobs. In this chapter, we talked about the three major types of cytoskeletal elements, each with its unique mechanism of formation and regulation to harness these structures to meet cellular needs.
Intermediate filaments, so called because they are intermediate in size, are the only nonpolar cytoskeletal element. This means that the two ends of the filament have no identifiable differences between them. Because they are nonpolar filaments, motor proteins don’t associate with them due to the lack of directional cues. These ropelike filaments provide tensile strength to help cells withstand mechanical strain.
Microtubules are the largest diameter cytoskeletal elements. These are hollow tubes formed from tubulin monomers, which themselves are made of an alpha and a beta-subunit. Assembly of microtubules is spontaneous, and these filaments will continuously assemble and disassemble based on a number of different factors. This dynamic instability allows microtubules to form and reform quickly, which makes them quite easy to rearrange. Microtubule-associated proteins (MAPs) are used to control when and where microtubules assemble and when they are disassembled. Microtubules are responsible for a variety of cellular processes, including vesicle trafficking, chromosomal segregation in mitosis, organelle positioning, and specialized motility structures like cilia and flagella.
Finally, actin filaments are the smallest diameter filament. Like microtubules, they undergo dynamic instability and are influenced by the binding of cellular proteins to influence stability of the filament as well as where and when these can form within a cell. These filaments are also polar; have a wide variety of proteins that control them, known as actin-binding proteins (ABPs); and associate with motor proteins. Actin filaments are responsible for functions like cellular locomotion, muscle contraction, cytoplasmic streaming, cell shape, and intracellular transport.
Review Questions
Note on usage of these questions: Some of these questions are designed to help you tease out important information within the text. Others are there to help you go beyond the text and begin to practice important skills that are required to be a successful cell biologist. We recommend using them as part of your study routine. We have found them to be especially useful as talking points to work through in group study sessions.
Topic 6.1: Overview of the Cytoskeleton and Intermediate Filaments
- List as many ways as you can think of that Intermediate filaments are the same and different from microtubules.
- List as many ways as you can think of that Intermediate filaments are the same and different from actin filaments.
- Describe the organization of intermediate filaments going from the level of single polypeptides to the microscopically visible filaments that are found in cells.
- Explain how the structure of intermediate filaments contributes to its strength.
- There are no known motor proteins that bind and move along intermediate filaments. Why might that be?
- How does the nuclear lamina help to organize the nucleus?
- Why do we say that intermediate filaments help to maintain cell shape?
- What is the purpose of desmosomes and hemidesmosomes? How do they help maintain the integrity of the tissues in which they are found?
- What might be the benefit of having different kinds of intermediate filaments, like vimentin, keratin and neurofilaments? Can you imagine some kind of advantage to this arrangement, especially when compared to things like actin and microtubules?
Topic 6.2: Microtubules and Dynamic Instability
- Explain how tubulin dimers interact noncovalently to make microtubules with a “plus” and a “minus” end.
- What is meant by polarity in microtubule structure? Explain what is meant by the “plus” and “minus” end of the microtubule. (Hint: It has nothing to do with charge or hydrophobicity.)
- What are nucleation sites, why are they needed, and what is their role in microtubule formation?
- What are microtubule organizing centers (MTOCs)? What do they do?
- What does dynamic instability mean? How is GTP involved?
- Explain how the different microtubule motor proteins work and how their movement relates to the polarity of the microtubule.
- Describe how microtubules are arranged in animal cells and how their arrangement contributes to the structure and function of the endomembrane system. How might motor proteins contribute?
- List the different types of microtubule associated proteins and explain their role in cellular function.
Topic 6.3: Actin Filaments
- Compare and contrast the polymerization of actin with that of microtubules.
- Compare and contrast the motor proteins associated with microtubules and actin microfilaments with regard to general properties, structure, and function.
- Explain how animal cell motility is dependent on the dynamic instability of actin filaments.
- Describe how associated proteins modify the function of both actin filaments and microtubules.
- Drugs are often used to disrupt normal cytoskeletal function in experiments. Explain how this might be used to learn about actin and/or microtubule function.
References
Kollman, J. M., Merdes, A., Mourey, L., & Agard, D. A. (2011). Microtubule nucleation by γ-tubulin complexes. Nature Reviews Molecular Cell Biology, 12, 709–721. https://doi.org/10.1038/nrm3209
Maggi, L., Mavroidis, M., Psarras, S., Capetanaki, Y., & Lattanzi, G. (2021). Skeletal and cardiac muscle disorders caused by mutations in genes encoding intermediate filament proteins. International Journal of Molecular Sciences, 22(8), article 8. https://doi.org/10.3390/ijms22084256
Mitchison, T., & Kirschner, M. (1984). Dynamic instability of microtubule growth. Nature, 312(5991), 237–242. https://doi.org/10.1038/312237a0
Prokocimer, M., Barkan, R., & Gruenbaum, Y. (2013). Hutchinson–Gilford progeria syndrome through the lens of transcription. Aging Cell, 12(4), 533–543. https://doi.org/10.1111/acel.12070
Roostalu, J., & Surrey, T. (2017). Microtubule nucleation: Beyond the template. Nature Reviews Molecular Cell Biology, 18, 702–710. https://doi.org/10.1038/nrm.2017.75
Walker, R. A., O’Brien, E. T., Pryer, N. K., Soboeiro, M. F., Erickson, H. P., & Salmon, E. D. (1988). Dynamic instability of individual microtubules analyzed by video light microscopy: Rate constants and transition frequencies. Journal of Cell Biology, 107, 1437–1448. https://rupress.org/jcb/article-pdf/107/4/1437/1461571/1437.pdf
Polymers of actin subunits that are part of the cell’s cytoskeletal system.
“Polymers of tubulin that form part of the cytoskeleton and provide structure and shape to eukaryotic cells.” Source: Microtubule. (2023, July 15). In Wikipedia, The Free Encyclopedia. https://en.wikipedia.org/wiki/Microtubule
A family of proteins that polymerize to form ropelike structures. These provide mechanical strength as part of the cytoskeletal system.
“Refers to the coexistence of assembly and disassembly at the ends of a microtubule. The microtubule can dynamically switch between growing and shrinking phases in this region.” Source: Microtubule. (2023, July 15). In Wikipedia, The Free Encyclopedia. https://en.wikipedia.org/wiki/Microtubule
“A specialized layer of cytoplasmic proteins on the inner face of the cell membrane.” Source: Cell cortex. (2023, February 12). In Wikipedia, The Free Encyclopedia. https://en.wikipedia.org/wiki/Cell_cortex
A specialized structure in Eukaryotic cells containing microtubules and a specialized MTOC called a basal body.
“A hairlike appendage that protrudes from certain plant and animal sperm cells, and from a wide range of microorganisms to provide motility.” Source: Flagellum. (2023, July 13). In Wikipedia, The Free Encyclopedia. https://en.wikipedia.org/wiki/Flagellum
“The flow of the cytoplasm inside the cell, driven by forces from the cytoskeleton.” Source: Cytoplasmic streaming. (2023, July 7). In Wikipedia, The Free Encyclopedia. https://en.wikipedia.org/wiki/Cytoplasmic_streaming
Composed of one unit. With a protein, this refers to having a single polypeptide chain.
A strand of repeating units called monomers.
“Made up of elongated or fibrous polypeptide chains which form filamentous and sheet-like structures.” Source: Fibrous protein. (2022, September 7). In Wikipedia, The Free Encyclopedia. https://en.wikipedia.org/wiki/Fibrous_protein
“The word dimer has roots meaning ‘two parts,’ di- + -mer. A protein dimer is a type of protein quaternary structure.” Source: Protein dimer. (2023, May 15). In Wikipedia, The Free Encyclopedia. https://en.wikipedia.org/wiki/Protein_dimer
Refers to two molecules that bind in a head-to-tail orientation.
A group of four proteins.
A cellular structure that assembles without input of energy.
A form of protein structure that is produced when two alpha helices wind around each other to produce a super coiled structure.
“A cell structure specialized for cell-to-cell adhesion.” Source: Desmosome. (2023, May 27). In Wikipedia, The Free Encyclopedia. https://en.wikipedia.org/wiki/Desmosome
“Very small stud-like structures found in keratinocytes of the epidermis of skin that attach to the extracellular matrix.” Source: Hemidesmosome. (2023, April 21). In Wikipedia, The Free Encyclopedia. https://en.wikipedia.org/wiki/Hemidesmosome
A family of proteins. Notably, alpha and beta tubulin dimers will polymerize into microtubules.
A single polymerized strand of microtubules. Thirteen such strands associate into a hollow tube-like structure.
The portion of the microtubule filament that grows faster and has a lower critical concentration. This is the end with beta tubulin exposed.
The portion of the microtubule filament that grows slower and has a higher critical concentration. This is the end with alpha tubulin exposed.
Refers to molecules that have a distinct endedness. If referring to electron distribution, molecules with unequal electron sharing are said to be polar. Extreme versions are ions. With regards to the cytoskeleton, the protein face that is exposed is distinct and refers to the polarity in these elements.
A beginning stage of a chemical reaction where the reaction proceeds slowly.
Can be thought of in three ways: the monomer concentration needed to initiate polymer formation, the monomer concentration at reaction equilibrium, and the concentration at which a polymer goes from shrinking to growth.
A buildup of GTP bound beta subunit accumulates at the end of a microtubule, protecting it from depolymerization (catastrophe).
“A structure found in eukaryotic cells from which microtubules emerge.” Source: Microtubule organizing center. (2023, March 23). In Wikipedia, The Free Encyclopedia. https://en.wikipedia.org/wiki/Microtubule_organizing_center
A microtubule organizing center (MTOC) in animal cells. It contains a pair of perpendicular centrioles.
A specialized tubulin network that acts as a nucleating site for microtubule growth.
A preformed structure that allows molecules in a reaction to be more favorably arranged. In regards to the cytoskeleton, nucleation sites include small preformed pieces of cytoskeleton. These make it easier for other cytoskeletal monomers to attach and thus speeds up the rate of reaction.
A cylindrical organelle at the center of a centrosome in animal cells.
A cellular process of nuclear division.
Networks of either actin or microtubules.
Proteins that bind to microtubule polymers. This prevents them from growing or shrinking.
Proteins that bind to cytoskeletal filaments and use energy to move along them.
A molecular motor that uses microtubules. Most kinesin motors move toward the plus end, but not all.
A molecular motor that uses microtubules. All known dynein motors move toward the minus end. Plants do not have dyneins and instead use minus-end directed kinesin to fulfill this role.
Refers to molecules that have one end of the molecule that is different from the other end. Most often, this refers to an uneven electron distribution, where extreme uneven distribution of electrons results in ionized molecules with a charge. Polar can also refer to shape differences on different ends of a molecule, as is the case with polar cytoskeletal elements.
Proteins that bind to actin (monomers or filaments) in vivo to modulate their function.
The monomeric actin subunit that is not attached to any other actin units.
An actin-binding protein that binds to actin filaments and promotes a new branched actin filament.
A molecular motor that uses actin filaments. Most myosin motors move toward the plus end, but not all.
The portion of the cell that is at the “front” with respect to the direction of movement.
“A cytoskeletal protein actin projection on the leading edge of the cell.” Source: Lamellipodium. (2022, November 28). In Wikipedia, The Free Encyclopedia. https://en.wikipedia.org/wiki/Lamellipodium