A New Nature
10 Super Ecosystems Last All Summer Long
Can We Future-Proof Ecosystems? Should We?
In Mrs. Swinton’s garden, it was always summer. The lovely almond trees stood about it in perpetual leaf. Monica Swinton plucked a saffron-colored rose and showed it to David.
—Brian Aldiss, “Supertoys Last All Summer Long”
On August 15, 1934, William Beebe and Otis Barton became the first people to dive to a depth of 3,028 feet (923 m)—a half mile below the surface of the Atlantic Ocean off the coast of Nonsuch Island, Bermuda. They did it in a hollow steel ball, outfitted with an air supply and a few windows, named the Bathysphere (Fig. 10.1). The Bathysphere was tightly tethered to a ship that lowered it down and up. Even that limited viewpoint provided an amazing glimpse into a world we had never seen before. As they dangled a few feet off the bottom of the abyss, Beebe and Barton might as well have been hovering over the surface of Mars. Beebe thought outer space was a good comparison:
the only other place comparable to these marvelous nether regions, must surely be naked space itself, out far beyond atmosphere, between the stars, where sunlight has no grip upon the dust and rubbish of planetary air, where the blackness of space, the shining planets, comets, suns, and stars must really be closely akin to the world of life as it appears to the eyes of an awed human being, in the open ocean, one half mile down.1
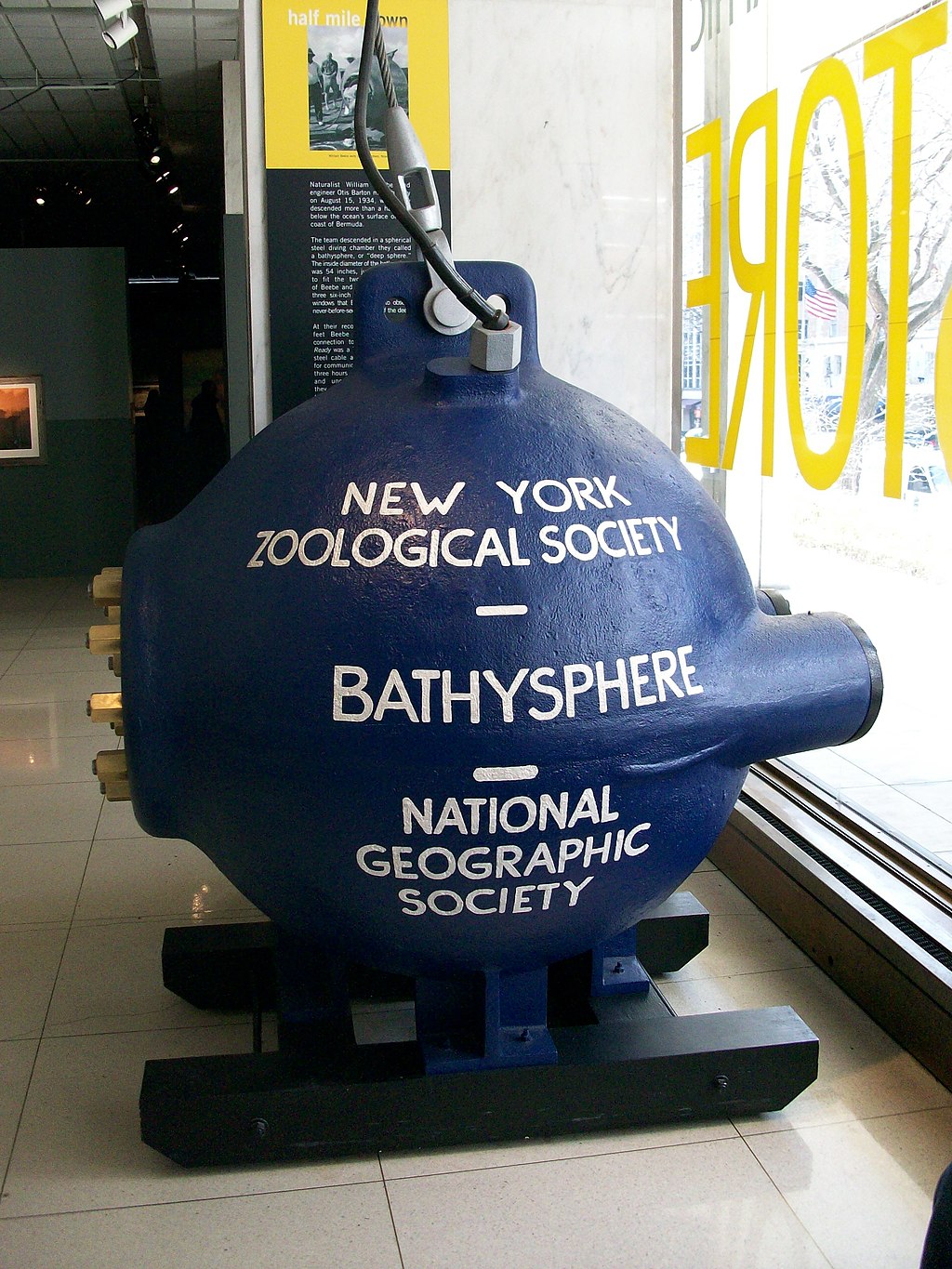
The record-setting dive was the culmination of a research effort that had started in 1928 when Barton convinced Beebe that he could design and build a submersible that would allow them to explore the deep ocean. Beebe was an ecologist and explorer with the New York Zoological Society who was keen on doing just that. After the Bathysphere was built, Beebe assembled a scientific team that arrived on Nonsuch in the spring of 1930. The team was notable not only for its pioneering scientific goal, but also for the fact that it included several women. At the time, women were routinely discouraged and excluded from science. When they were part of scientific teams, they often served only menial support roles despite their skill and experience. In contrast, the women on the Nonsuch expedition held key positions. Gloria Hollister was an expert in fish osteology who developed innovative ways of preserving fish specimens. In 1934, she reached a depth of 1,208 feet (368 m) in the Bathysphere. Jocelyn Crane was fresh out of college at the time, but her work on the expedition propelled her career as one of the world experts on crustaceans. She went on to become the director of the New York Zoological Society’s Department of Tropical Research. Else Bostelmann was an artist who made technical drawings of the observations and specimens. She would paint underwater scenes firsthand while wearing a diving suit.2
Over several years, the team made repeated dives in the Bathysphere and documented an amazing menagerie of bizarre new life. On one dive, Beebe described a fish that had never been seen before—and it has never been seen since:
A single line of strong lights, pale bluish, was strung down the body. The usual second line was quite absent. The eyes were very large, even for the great length of the fish. The undershot jaw was armed with numerous fangs which were illumined either by mucus or indirect internal lights. . . . There were two long tentacles, hanging down from the body, each tipped with a pair of separate, luminous bodies, the upper reddish, the lower one blue. These twitched and jerked along beneath the fish, one undoubtedly arising from the chin, and the other far back near the tail.3
The excitement generated by the Nonsuch expedition helped launch a golden age of deep-sea exploration that yielded discoveries of equally strange wonders such as hydrothermal vent ecosystems based on chemosynthesis instead of photosynthesis,4 and fish that can live at a depth of 26,496 feet (8,076 m) near the bottom of the Mariana Trench.5 Advances in technology promise yet more discoveries. We now have autonomous submersibles that can explore the ocean for weeks at a time.6
In some ways, however, the Nonsuch expedition epitomized an approach to studying the natural world that was beginning to radically change. With even the remotest corners of the world now accessible, scientists weren’t satisfied with just cataloging biodiversity and arranging it neatly into cabinets of curiosity. They began to ask more pointed questions about how ecological systems were constructed and how they functioned. Researchers also began adapting new technologies, not only to help explore little-traveled parts of the world, but also to design experiments and test hypothesis about life. These new approaches blossomed after World War II. Just two decades after the last dive of the Bathysphere, scientists would publish papers describing the double-helix structure of DNA (deoxyribonucleic acid),7 the flow of carbon and energy through a coral reef,8 and the population dynamics of introduced non-native animals and plants.9
At the same time, the increasingly rapid changes affecting the biosphere spurred research aimed at figuring out how to save biodiversity from destruction. We used our emerging understanding of ecology and evolution to develop conservation strategies and make policy recommendations. By the end of the twentieth century, the field of conservation biology had developed a range of tools and approaches for reducing and mitigating the negative effects that our actions were having on ecosystems. Historically, these efforts have been conservative in that they sought to minimize the changes we were causing and to repair any damage. Our growing understanding and use of the deep ocean are good examples. The same technologies that gave researchers access to the deep ocean also provided opportunities for commercial exploitation such as oil and gas extraction and deep-sea mining. Researchers realized that these activities could potentially threaten the unique and complex ecosystems that we were just beginning to understand.10 We have applied our limited ecological knowledge of these systems to help create deep-ocean parks that protect unique hotspots of biodiversity,11 to design best practices that limit the environmental impact of activities such as deep-sea mining,12 and to restore deep-sea habitats that have been degraded.13
Our understanding of Earth’s ecology as well as our ability to alter it have accelerated in the twenty-first century. As the previous chapters in this book describe, we now influence nearly every aspect of the Earth System. Much of that influence is pervasive and has seemingly become an intrinsic aspect of Earth’s natural history. The Mariana Trench, perhaps the most remote and unpopulated place on Earth, is now as polluted with industrial toxins and plastics as are rivers flowing through heavily industrialized regions in China.14 Our social and political institutions often seem slow to act or, in some cases, incapable of acting to develop policies that could mitigate environmental degradation.
As a result, conservation became more proactive. The field of restoration ecology arose to develop ways of repairing damaged ecosystems (Chap. 9). But some scientists argue that we now need to go beyond repair and restoration. We may need to design new patterns of biodiversity and direct evolutionary trajectories. We may need to design Anthropocene-adapted ecosystems. In this chapter, I briefly outline some of the approaches that have been proposed for doing that. These ideas make use of a range of new tools that allow us to manipulate the basic elements of biodiversity.
10.1 The Biotechnology Revolution
Section 10.1: The Biotechnology Revolution
Biotechnology is a rapidly evolving field that increasingly influences many aspects of society; biodiversity conservation is no exception. Here, I briefly describe some of the main biotechnology tools that are being used (or considered) for conservation. I also give a few examples of their applications in the following sections.
Genomics
One set of tools is based on our increasing ability to sequence the entire genome of a species quickly and inexpensively. In 2001, it cost $100 million to sequence a genome. In 2020, the cost hovered around $600, and one company said it could do it for you for $100—coffee and doughnuts in the waiting room.15 We can couple genome sequencing with other techniques such as genotyping by sequencing to describe how individuals differ in their genetic makeup.
Functional genomics are various approaches for understanding how an organisms’ entire genome interacts with the environment to influence its ecology and how it functions. Until recently, our ability to understand how genetic variation is related to phenotypic traits was constrained by the long, expensive, and technically difficult process of identifying genes, understanding what functional roles those genes played, and understanding how those genetic functions got translated into phenotypes. The ability to quickly sequence entire genomes has allowed us to speed up this process.
Genome-wide association studies attempt to identify genomic variation that is associated with a particular phenotype. One basic approach is to associate patterns of genomic variation across individuals or populations with variation in the environmental conditions that those individuals or populations experience. We can ask a question like, How do the genomes of populations in a dry part of a species’ range differ from those of populations in a wet part of the range? Any differences in their genomes might be related to—that is, associated with—adaptions to their local conditions. We can also directly compare the genomes of organisms that we know differ in a trait. For instance, we can compare the genomes of individuals that are tolerant of high temperatures with those that aren’t to identify genetic differences that are associated with the heat tolerance trait. Genome-wide association does not initially identify specific genes or the underlying mechanisms driving a trait, but for many applications, that is not all that important. In fact, in many cases, looking for a critical gene or two is not the best approach. Phenotypic traits are often determined by many genes interacting with each other and the environment in complex ways. In genetics jargon, these are called quantitative traits. Genome-wide association studies give us a big-picture view of the entire genome so that we can more easily see all the different parts that influence quantitative traits.
We can use genome-wide association studies to identify genetic markers that are proxy signposts for parts of the genome that are associated with a quantitative trait. These markers are called quantitative trait loci (or QTLs, in the acronym-enamored world of biotechnology). An important application of quantitative trait loci is to quickly screen individuals for traits without having to observe the organisms expressing the traits directly. That might not sound all that exciting, but it is an extremely useful tool. Most of the traits that we are interested in involve how organisms respond to and interact with their environment, and in particular how they respond to stressful changes in environmental conditions, such as drought tolerance, cold hardiness, pollution tolerance, disease resistance. Directly observing these traits in individuals or populations is usually time consuming, expensive, and in many cases not all that feasible. Imagine trying to evaluate how tolerant 50-m-tall Douglas fir trees (Pseudotsuga menziesii) are to 100-year drought events. If we have identified quantitative trait loci that are associated with tolerance to drought conditions, we can easily take tissue samples from trees and quickly screen them for the presence of the drought tolerance loci.
The growing amount of genomic information has many applications in the service of biodiversity conservation. One of the most fundamental is that we are developing a more detailed picture of the genetic component of biodiversity, not only within individuals and populations but across large landscapes as well.16 Until recently, the best that we could do was to describe how the frequency of a few genes or neutral stretches of nucleotide sequences differed among populations. Plus, because the analyses were expensive, most studies could only describe variation across a handful of populations. But the genomic revolution is allowing us to describe how entire genomes vary among individuals and across populations. Declining costs are also allowing researchers to describe that variation across large, spatially complex landscapes. For instance, affordable, portable DNA sequencers allow scientists with even modest research budgets to monitor genetic diversity across many individuals and populations.17
Seeing patterns of genetic variation from the perspective of the entire genome allows us to better identify genetically unique populations that we might want to prioritize for conservation. If we have information about how the genomic variation relates to specific traits, we can assess the risk faced by different populations and predict how the distribution of populations will change. An example comes from studies that have assessed the climate risk faced by Eucalyptus species in Australia. The studies use genome-wide association analyses to identify genomic differences that are indicators of adaptation to particular local environmental conditions. The studies have found that Eucalyptus populations vary considerably in their potential to adapt to changing climate. For example, many populations of Eucalyptus tricarpa are adapted to cool and wet conditions. Predicted climate change toward warmer and drier conditions will potentially be outside the adaptive capacity of these populations, leaving the species as a whole in a precarious condition.18 That type of information can be used to plan conservation strategies. Populations of another Eucalyptus species, E. melliodora, similarly vary in their adaptive capacity to adjust to predicted climate change. Some populations have genomes that seem to be better suited for future conditions than others (Fig. 10.2). Conservation efforts could try to increase gene flow between the least and most climate vulnerable populations in hopes of facilitating the spread of advantageous alleles and improving the climate resilience of the more vulnerable populations.19
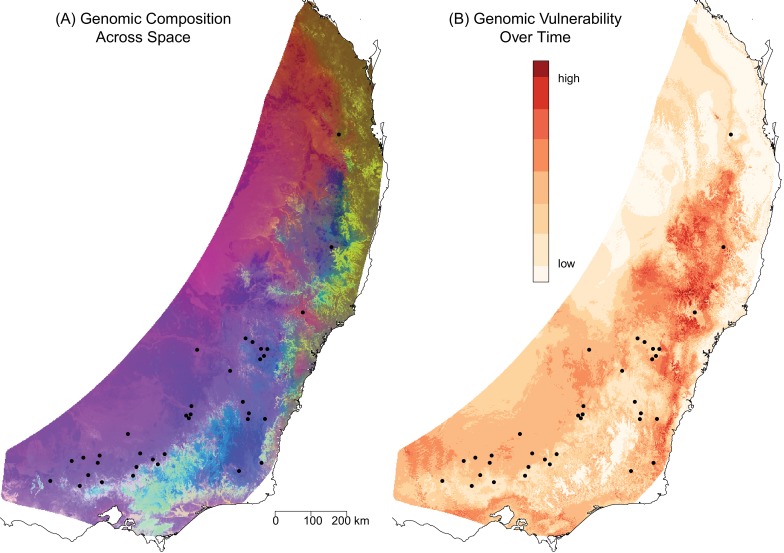
Marker-assisted selection is another applied use of genomics. Marker-assisted selection uses genomic tools to help facilitate selective breeding. Selective breeding is laborious. It starts with raising individuals, screening them for traits of interest (which often involves manipulations like exposing them to heat or inoculating them with a virus), then selecting the promising-looking ones. The promising ones are then used to make crosses, after which the whole screening process is repeated on the offspring. That process could go on for several generations. Sometimes just growing individuals until they reach an age when they can be screened and crossed takes an excruciatingly long time—such as with many tree species. But the process can be rapidly sped up if we develop genetic markers that are associated with the traits we are interested in. For instance, a set of young tree saplings can be assessed for the presence of virus-resistance genetic markers in the fraction of the time and expense it would take to screen the phenotypic trait directly. Selective breeding has not historically been a tool used for conservation. But it is increasingly being considered as a way to help develop populations that will be more tolerant of Anthropocene conditions. I describe a couple examples in the section on assisted evolution below.
Epigenomics
Acclimatization is the physiological process by which individuals adjust to changing environmental conditions. For instance, humans have a modest ability to acclimatize to low-oxygen conditions, which is why climbers usually spend at least a few days hanging out at base camp before they attempt a big climb. We used to think that the changes associated with acclimatization were relatively short term and not passed down from parent to offspring. For instance, an avid climber would not have children who were born pre-acclimatized to high altitudes. But we have discovered that at least some acclimatization changes can be passed on to offspring through a range of processes collectively called epigenetics. Broadly, these involve nongenetic changes to cellular mechanisms that regulate gene expression.
One epigenetic mechanism is DNA methylation, which is the addition of methyl groups to DNA. The presence (or absence) of methyl groups strongly influences gene expression. A number of environmental factors affect patterns of methylation (as well as the other epigenetic mechanisms), and these changes can sometimes be passed along to offspring. For instance, toward the end of World War II, the Nazis imposed a blockade on the Netherlands that plunged the country into famine. Women who were pregnant during this time were particularly affected by the shortage of food, and it seems that they passed on a pattern of stress-induced DNA methylation to their children. Years later, when the children grew to middle age, they suffered higher rates of maladies such as diabetes and obesity than children whose parents had not experienced famine.20 Not only can epigenetic changes persist for a few generations, but they can also be retained by individuals for a long time. In some cases, individuals that have been exposed to stresses in the past respond more effectively if they experience the same stress again in the future. For example, communities of people living at high elevations have unique mountain-adapted physiological traits. Those adaptations probably reflect both changes to their genes as well as epigenetic mechanisms.21
In some cases, populations may develop epigenetic adaptations to environmental conditions before they develop genetic adaptations. An example comes from invasive populations of the asexual New Zealand freshwater snail (Potamopyrgus antipodarum) that are spreading in the western United States. The snail has invaded both stream and lake habitats, and in just 30 years, populations living in streams have developed larger shell apertures and foot muscles than those living in lakes. This difference appears to be adaptive; bigger feet provide better grip on rocks in fast-moving currents. But there is little genetic variation between stream and lake populations, suggesting that direct differential selection of alleles hasn’t taken place. Stream and lake populations do have significantly different patterns of genome-wide DNA methylation, suggesting that morphological differences reflect epigenetics.22
We can potentially exploit epigenetic mechanisms as tools for biodiversity conservation. One idea is to develop epigenetic markers similar to the genomic markers that are based on DNA sequences. We could use this epigenome information to better understand the environmental potential of individuals and populations.23 For instance, we could identify populations or individuals that are best (or least) able to adjust to climate change in part by looking at their DNA methylation signatures. We might also manipulate the epigenetics of populations to help acclimatize them to the stressful conditions associated with climate change. That could buy important time for other ecological and evolutionary processes such as range shifts and natural selection to make longer-lasting adjustments, or for us to reduce our climate impact.
Genetic Engineering
Genetic engineering involves various methods for directly manipulating genomes, often by inserting or deleting genes or by editing sequences of genetic code. Many of these techniques develop recombinant DNA constructs that combine DNA sequences from multiple sources, which are then inserted into a recipient genome. The resulting recombinant genomes can contain genes from distantly related taxa, creating transgenic organisms. A form of genetic engineering called genome editing includes a range of techniques that allow relatively precise alterations to genetic sequences that do not necessarily involve the insertion of genes from distantly related taxa. The most common of these tools is the CRISPR-Cas9 system. (CRISPR stands for clustered regularly interspaced short palindromic repeats; see the Additional Resources for an overview).
Much like selective breeding, genetic engineering techniques have not been broadly used as conservation tools. This is beginning to change, however. The “copy-and-paste” aspects of genetic engineering tools have a number of applications for directly preserving (or even resurrecting) genetic diversity (see the section on cloning below). Another potential application is the development of novel traits or functions that will allow populations to survive the Anthropocene. For example, most of Hawaii’s native birds are now restricted to high elevations, in part because of habitat conversion at low elevations. Another major factor is the presence of avian malaria, a mosquito-borne disease that has contributed to severe population declines and extinctions. The main vector of avian malaria in Hawaii is the non-native southern house mosquito (Culex quinquefasciatus), whose range is currently restricted to low elevations. But climate change is expected to allow the mosquito to move into elevations that currently provide malaria-free refuges. A few of Hawaii’s native bird species have evolved tolerance to avian malaria. We could use genomic approaches to help identify the genetic basis for that tolerance. We could then potentially bestow tolerance on other more susceptible species using gene editing tools.24
But getting any novel traits that we might develop to spread in wild populations is not straightforward. Our current approach is to rear individuals with desirable traits in controlled environments such as a nursery, release them into the wild, and hope that they establish populations. For instance, we could develop and release forms of an endangered alpine plant that are more heat tolerant than the wild type as a way to help the species survive the climate-change-induced warming of its habitat. Presumably, the more heat-tolerant individuals would have an advantage, and they should establish wild populations that grow and spread. But this approach can be expensive and logistically challenging even for rare species with small populations and restricted ranges. In addition, it is difficult to rear individuals in captivity that represent the full genetic diversity reflected in wild populations. If we use captive-reared individuals to replace wild populations, we potentially will lose evolved adaptions that allow populations to survive the diverse range of local conditions. A more ideal solution would be to seed beneficial genes into existing populations and have them spread on their own via sexual reproduction.
The rules of inheritance create a significant roadblock to this solution. As a general rule, each different allele of a gene has an equal chance of getting transmitted to offspring. So, in diploid organisms, alleles have a 50% chance of getting transmitted to the next generation. That half chance of not being passed on creates a significant constraint if we want to introduce a new allele or gene into a wild population. The number of individuals that we can release is usually tiny relative to the size of wild populations. It is therefore likely that the novel genes they contain will go extinct by random chance. Even if the genes manage to avoid random extinction, the genes will be slow to spread in the population unless they confer a strong selective advantage. That may not always be the case, even if we think the genes are advantageous. For instance, we might want to spread a gene that conferred resistance to periodic severe droughts whose frequency and severity are expected to increase because of climate change. It could be years before a severe drought happened, and in the meantime, the severe drought genes wouldn’t have any selective advantage.
Gene drives are a potential way around this problem (Fig. 10.3). Gene drives are a common natural phenomenon involving several different mechanisms that increase the probability that a gene will be transmitted to offspring. There is evidence that natural gene drives have played important roles in shaping the evolution of genomes.25 We have developed our own engineered versions of gene drives; the most common one to date is based on the CRISPR-Cas9 genome editing system. Gene drives could be used to efficiently spread beneficial genes through populations. But they can also be used to spread genes that are deleterious and would otherwise be selected against. It is that application that has gotten the most attention so far, mostly in the realm of pest management. One conservation application is in the management of invasive species.
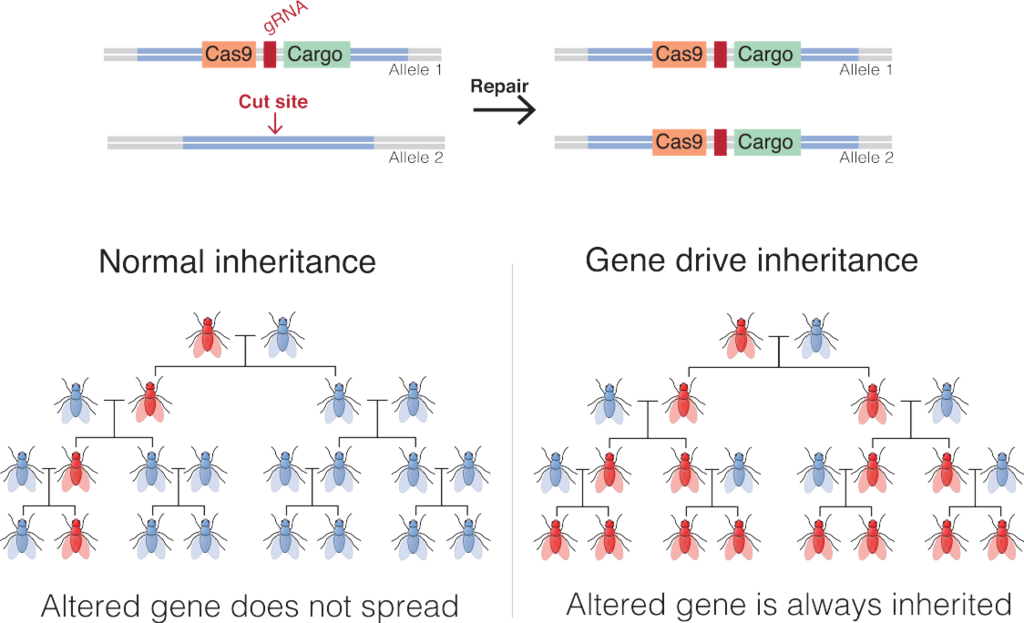
Gene drives could be used to spread deleterious genes through invasive populations that would reduce their population size or even eradicate them altogether. An example that has gotten a fair bit of consideration is the use of gene drives to eradicate invasive rodents from islands. Invasive rodents, like other small mammals, have had a negative impact on island biodiversity (see Section 9.3), and consequently, rodent eradication has become a conservation priority for many islands. These efforts currently involve the aerial broadcast of anticoagulant rodenticides, which is costly and can have nontarget effects on ecosystems. Several gene-drive-based systems have been proposed as alternatives. One involves exploiting a naturally occurring gene drive in mice called the t-haplotype. Males that are homozygous for the t-haplotype either die before birth or are sterile. Despite that clear disadvantage, males that are heterozygous for the t-haplotype pass it on to their offspring at rates up to 95%. Researchers can use genetic engineering tools to hitch the t-haplotype gene drive to the Sry gene that determines male sexual characteristics. In concept, that gene combination would spread through the population, creating offspring that were more than 90% phenotypically male, and that would eventually lead to a nonviable population that was almost entirely phenotypically male.26
Not surprisingly, gene drives are controversial.27 You don’t have to be overly cautious to see the various ways that things could go wrong from ecological, social, and ethical perspectives. One broad technical concern is that the genes we introduce via a gene drive, along with the traits they bestow, could spread to populations that we had not intended. For example, a deleterious gene introduced to eradicate an invasive population on an island could spread to native populations of the same species via natural dispersal or unintentional human transport. In a worst-case scenario, we could even cause a species to go extinct. Researchers are developing designs that allow more precise control and include more inherent safeguards. In the case of island rodents, for example, gene drives could be engineered that will only spread through populations that have a unique genomic signature. Most invasive island rodent populations have evolved unique genomic signatures that differentiate them from mainland populations. A gene drive could be tuned using those unique signatures so that it would not work if it was accidently introduced off the island.28
Cloning
There are a variety of different tools and techniques that fall under the broad umbrella of cloning. Some are old, such as the asexual propagation of plants using grafting or cuttings. We have also developed several newer approaches.
Molecular Cloning
Molecular cloning involves the range of techniques for assembling recombinant DNA molecules and then orchestrating their insertion and replication in a recipient cell. It is one of the core set of tools in the genetic engineering toolbox.
Reproductive Cloning
Reproductive cloning is used to create copies of entire multicellular organisms. The main approach inserts a nucleus from a somatic cell into an egg cell that has had its own nucleus removed. We have figured out how to get the transformed egg cell to start dividing. We can then transplant the embryo into a surrogate using in vitro fertilization. The surrogate will then give birth to an individual that is genetically identical to the somatic cell we started out with. In 1996, a sheep named Dolly became the first mammal cloned from an adult cell.29 Therapeutic cloning is similar to reproductive cloning except that researchers use the transformed egg to grow stem cells. The technique is primarily used for basic research at the moment, but stem cells can be coaxed to develop into different cell types and tissues, which at least in concept can be used in medical applications such as growing replacement organs that won’t suffer from transplant rejection.
Tissue Culture
Tissue culture is a form of cloning that does not involve direct manipulation of genetic material. It includes a broad range of techniques for growing cells under sterile conditions in a nutrient medium. The composition of the nutrient medium can be tweaked to coax particular growth patterns. The most impressive results are achieved in plants. Individuals of many plant species can be readily cloned in tissue culture through the orchestrated use of plant hormones. A wide variety of tissues and cell types can be used as the starting source material, even including somatic cells in a process called somatic embryogenesis. Plant tissue culture (also called micropropagation) is commonly used to commercially propagate large numbers of genetically identical individuals. Many of the ornamental plants you see at garden centers have been grown, at least in part, using tissue culture.
Producing an endless supply of genetically identical individuals is typically not a goal of biodiversity conservation. Cloning does have some potential conservation applications, however. One of them is using tissue culture to help conserve biodiversity in ex situ conservation. Ex situ conservation encompasses the various ways of preserving individuals outside of the wild—that is, “off-site.” These include seed banks, botanical gardens, and captive breeding programs. Tissue culture is one of the tools used to maintain archive copies of genetic resources in these facilities. It can be an effective and efficient long-term storage solution, particularly when combined with cryopreservation, or preserving viable tissue in cold storage. Again, plant tissues are generally more amenable to being frozen and then reconstituted into fully functional organisms than are animal tissues (Fig. 10.4).
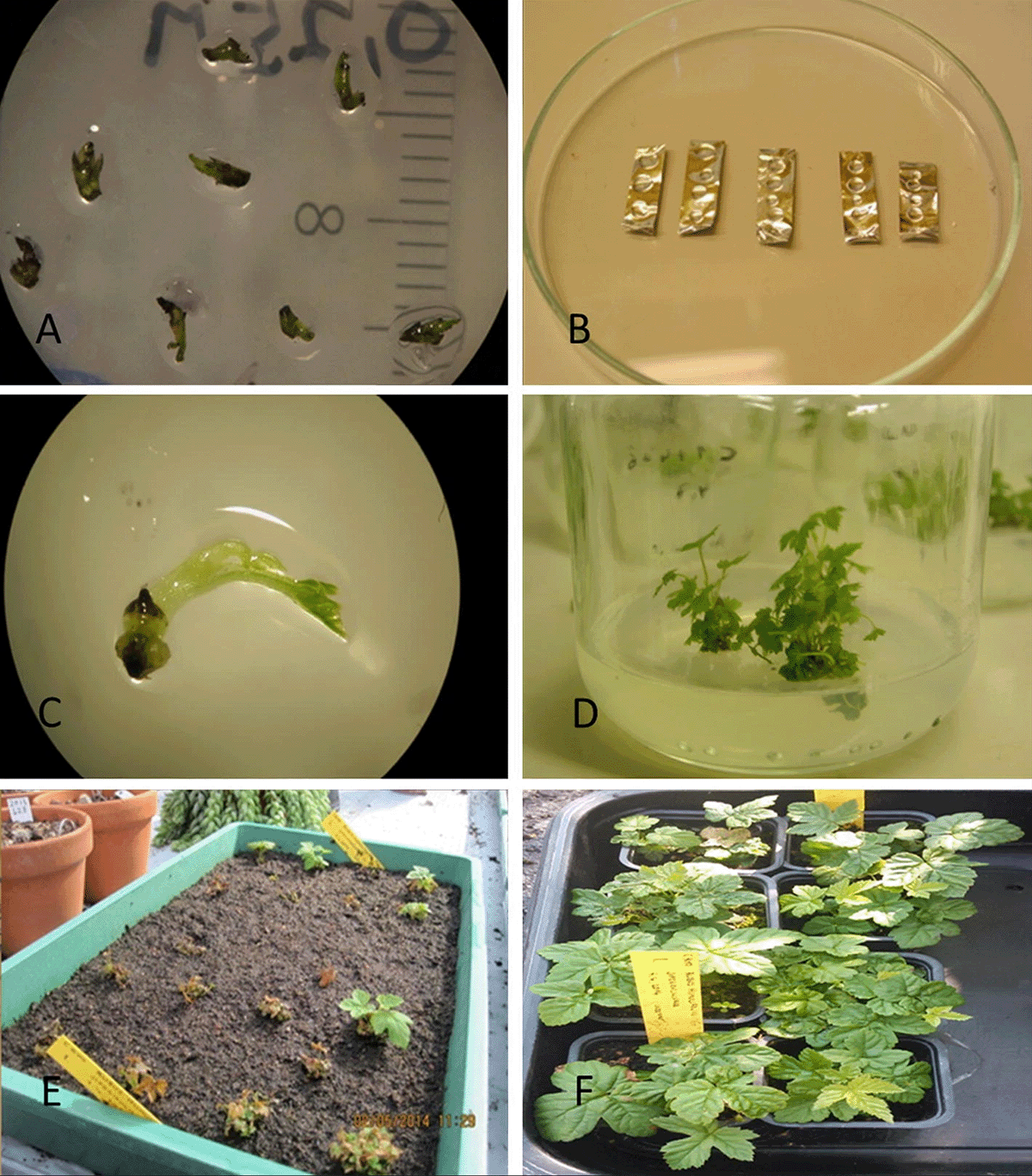
Another conservation use of cloning is to increase the population sizes of critically endangered species. Critically endangered populations often suffer low reproductive success both in the wild and in captivity. In addition, their low numbers make other methods of propagation such as the mass collection of wild seed risky. In the most extreme cases, there may only be a single individual left in the world. That was the case for Hāhā (Cyanea pinnatifida), a plant species endemic to Oahu, Hawaii. In the early 1990s, there was only a single individual known in the wild. Two side shoots were collected and used to propagate clones in tissue culture. Some of these clones were eventually planted in botanical gardens, and some were used to establish small populations in the wild. Those wild populations have yet to successfully recruit new individuals, but they do regularly produce seed (Fig. 10.5) that is added to the ex situ conservation bank.30
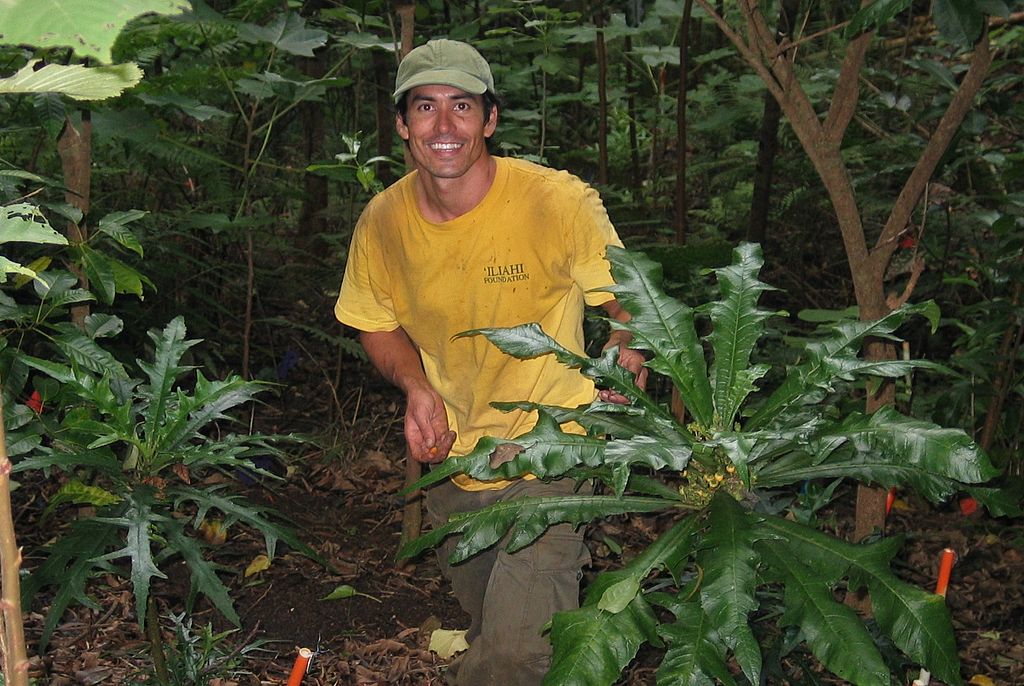
Reproductive cloning can be used in last-ditch efforts to save critically endangered animals, and clones of several endangered animal species have been produced.31 Cloning has even been used to resurrect a genetic line that had gone extinct. In 2002, a baby bucardo (Capra pyrenaica pyrenaica) was born to a domestic goat (Capra hircus). Researchers used preserved cells from the last living bucardo named Celia, who had died in 2000. The baby burcardo lived only a few hours, illustrating the significant technical issues that still constrain animal cloning. Currently, animal cloning is used only experimentally or as part of an effort to preserve the germplasm in ex situ conservation. New approaches that combine genetic engineering, reproductive cloning, and micropropagation techniques could yield new potential conservation applications, however. I mention a few possibilities in Section 10.2.
10.2 Introductions and Assisted Colonization
Section 10.2: Introductions and Assisted Colonization
Introducing populations and assisting their movement across landscapes are general approaches that could help conserve biodiversity and critical ecological relationships. All of these ideas further blur the distinction between our management of domesticated ecosystems and of wildlands. Some aspects of these approaches have been part of the conservation toolbox for some time. Other aspects remain largely experimental or wholly untested ideas, but they could become more prominent tools for conservation in the future.
Reintroducing Populations
Introducing populations of animals, plants, and microbes has long been an approach used in conservation and ecological restoration. Unlike our intentional and unintentional movement of non-native populations across biogeographic boundaries, the goal of conservation-oriented introductions is to reintroduce populations that had previously existed at a location during the recent past. This commonly involves using nearby populations as the source pool for reintroductions. As mentioned in Section 9.2, great pains are taken to ensure that the phenotypic and genetic characteristics of the reintroduced populations match as closely as possible those that previously existed at the site or that currently exist at nearby reference sites. In rare cases, local populations can be salvaged prior to a disturbance and then reintroduced to the same location, thus preserving much of the original local diversity. That technique was used to supply some of the plants for the residential landscape in Figure 8.15Figure 8.15. A residential landscape without the ubiquitous lawn. In contrast to the narrow goals and severe constraints facing designers of agricultural systems, urban landscape designers typically have much more flexibility. This landscape in Tumalo, Oregon, was designed to not need irrigation or other inputs despite being in relatively dry central Oregon. The designers achieved the low resource use by using regionally native plant species adapted to the local climate, including the microbial community of native soils to help regulate plant resource uptake and recycling, spatially combining species with complementary resource use in ways that mimic patterns in natural vegetation, and capturing rainfall on-site using a water feature. The design met the aesthetic goals for the homeowners as well as provided a range of other ecological functions, such as providing animal habitat. Source: courtesy of Richard Martinson.
Sometimes, populations of a species have been extirpated over a large area or the overall number of individuals has gotten perilously close to the edge of extinction. In these cases, population reintroductions are done using individuals sourced from distant populations or from individuals in ex situ conservation programs. When reintroduced populations are derived from distant or ex situ sources, it can be challenging to match the genetic or phenotypic composition of past local populations. We may not know what the past population characteristics were like. For example, the Eurasian beaver (Castor fiber) used to be common and widespread across Eurasia, but overhunting and habitat loss have caused a dramatic decline. They were extirpated from the United Kingdom in the sixteenth century, and by the twentieth century, there were only an estimated 1,200 individuals left in the rest of Eurasia. In 2013, a beaver family mysteriously reappeared on the River Otter in Devon, in southwestern England. No one knows how they got there; one possibility is that rogue conservationists were responsible.
The government originally wanted to remove the beavers, in part over concerns that they would damage crops and create flooding and erosion risks. The beavers were allowed to stay on for a five-year trial period. Over that time, the beaver population grew, and local landowners successfully adapted to their presence. In 2020, the government allowed the beavers to remain permanently.32 The success of this unplanned introduction spurred hopes that a larger-scale program could return beavers to all of Britain. But choosing genetically appropriate source populations is not a straightforward task. There aren’t any beavers living in the United Kingdom that can help us understand the genetic patterns of the historic populations, but we do have old Pleistocene beaver bones. Genetic analysis of ancient DNA from bones found in caves across Europe suggest that UK beavers were part of a large, evenly mixed population that was rapidly expanding across western Europe as glaciers retreated.
So, despite their relative isolation, the ancient UK beavers were not genetically distinct from their continental cousins, and there was not much genetic differentiation among populations across all of Europe. But that evenly mixed population genetic structure has changed dramatically. The genetic composition of all the remnant Eurasian populations has been strongly altered by the bottlenecks and isolation associated with the dramatic decline of the species. Populations across Europe are now much more genetically distinct from each other, and none is a clear genetic analog to the original UK beavers.33
In extreme cases, there is hardly any population-level diversity left, and all we can do is try to stop any further loss of the genetic diversity in the genomes of the remaining individuals. Efforts to save the California condor (Gymnogyps californianus) from extinction are a good example. In 1982, there were 22 California condors left in the world. They were all captured, and 14 were used in a captive breeding program that has been the source for reintroducing individuals to the wild. As of March 2020, there were 337 birds in the wild.34 Genome sequencing shows evidence of inbreeding, both from the recent crash of populations as well as from previous genetic bottlenecks in the more distant past. Given how few individuals are left, however, the remaining ones have a surprisingly high level of genetic diversity across their genomes. That probably reflects the fact that California condors had a huge range that stretched across much of North America, and there was probably significant gene flow between populations. The remaining individual genomes reflect that genetic interchange, which suggests that the recovering populations are in a good position to avoid the worst effects of inbreeding.35 We can help prevent that from happening by monitoring the genetic composition of the recovering populations and taking mitigating actions if there are signs of inbreeding. We have nevertheless lost much of the genetic differences among populations that once existed.36 The genetic composition of California condors living in Florida and Western Canada were likely quite different, with at least some of those differences reflecting adaptations to their respective local environments. That genetic history has largely been lost.
The lack of a detailed genetic past forces—and frees—us to think more about the genetic future. We are also beginning to think more creatively about the population genetics of reintroduced and translocated populations even in cases where there is still considerable wild genetic diversity left. For example, we could design the genetic and phenotypic composition of translocated populations in ways that we think will maximize their survival and growth. Having information about the functional genomics of a species would help us do that. In the case of UK beaver introductions, we could use individuals that have cold tolerance quantitative traits to found populations in northern Scotland, and individuals that have pollution tolerance quantitative traits to found populations in the urbanized British midlands.
Introducing Non-Native Populations
An even more provocative idea is to intentionally introduce taxa that have no close evolutionary history to a region. The main reason for doing this is to reintroduce ecological functions that were provided by now-extirpated native species. The idea is often associated with the conservation concept known as rewilding. Broadly, rewilding seeks to restore complex suites of ecological functions and relationships across large landscapes. I describe rewilding as an approach for ecologically intensifying agricultural landscapes in Chapter 7. A common aspect of many rewilding schemes is restoring populations of large animals (megafauna), particularly large herbivores and apex predators. The reasoning behind this approach is that megafauna often play disproportionately large functional roles in ecosystems. These include being important seed dispersers, creating disturbance patterns that promote high levels of plant diversity, influencing biogeochemical flows, and being important nodes in food webs.37 Their extirpation from regions has been one important driver of ecosystem change during the Anthropocene. Some argue that the ecological roles performed by these types of species are so important that we shouldn’t be hung up on the specifics of genetic identity or pedigree when trying to conserve or restore them. Instead, we should focus on conserving or restoring ecological functions—the functional components of biodiversity. In a 2006 article that was perhaps more rhetorical device than a serious call for action, a group of scientists argued that we should introduce free-roaming populations of non-native mammals to North America such as Asian elephants (Elephas maximus), lions (Panthera leo), and cheetahs (Acinonyx jubatus).38 Their reasoning was based on the fact that ecological analogs of these species went extinct in North America at the end of the Pleistocene (see Figure 1.5Figure 1.5. FIX. Source: ME. ). Introducing extant ecological analogs would help restore some of the functions and biodiversity that were lost at the end of the Pleistocene.
While that idea has yet to go beyond the second beer at conservation biology meetings, there have been a few more modest actual examples. One comes from conservation efforts on the Mascarene Islands, which make up the islands belonging to the Republic of Mauritius and the French department of Réunion. The islands used to be home to five endemic species of giant tortoises in the genus Cylindraspis, all of which had gone extinct by the early nineteenth century, primarily as a result of human activities such as overhunting (Fig. 10.6). The tortoises were the main herbivores on the islands, as well as important seed dispersers for many fleshy, fruited plant species. In an effort to restore the ecological functions that were lost when the endemic species went extinct, managers have introduced two species of non-native tortoises that are ecological analogs of the extinct species: the Aldabra giant tortoise (Aldabrachelys gigantea) and the radiated tortoise (Astrochelys radiata).39 Early results of the introductions are promising, with the introduced tortoises dispersing seeds of several native species and selectively grazing several non-native invasive plant species.40
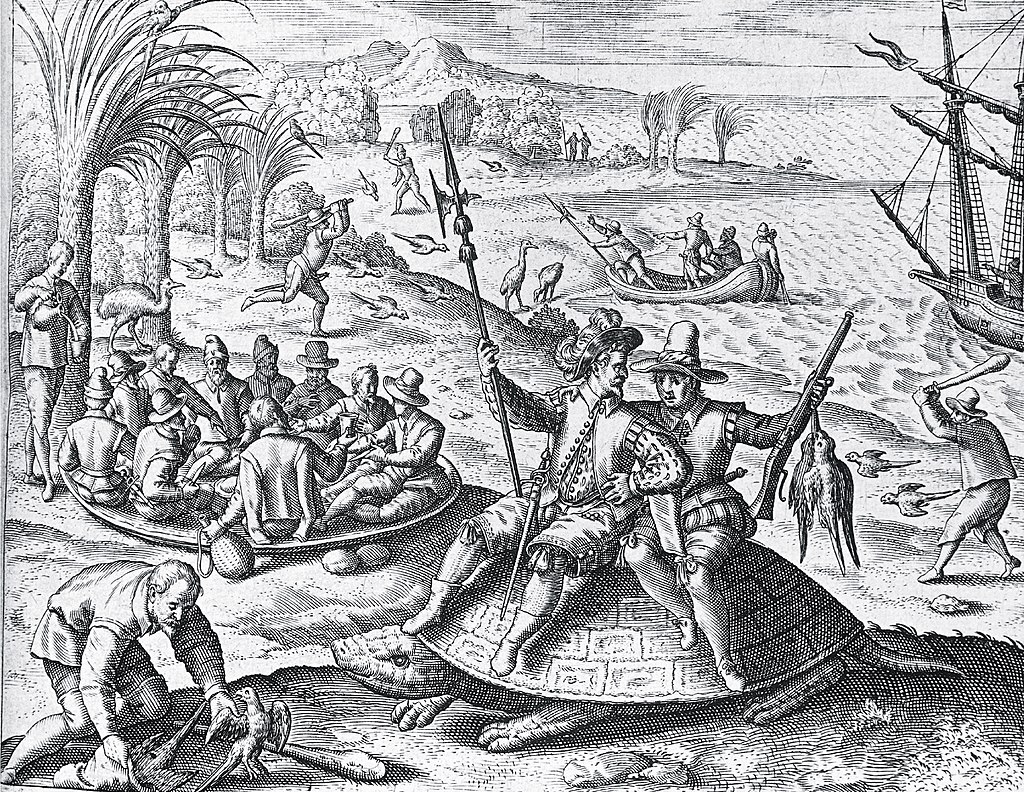
Another potential goal of introducing non-native populations is to help conserve species that are threatened with extinction. Introducing populations of threatened or endangered species to regions where they are non-native could provide insurance in case the few small populations in the native range go extinct—sort of like having off-site backups of your important computer files. The new introduced range might also have less of the issues that are causing the species to decline.
Assisted Colonization
Climate change is expected to shift the intrinsic ranges of species, and it is likely that many of them will not be able to adjust quickly enough to keep pace. Factors such as physical barriers to movement (both natural and human made) and the speed of climate change relative to a species’ innate dispersal ability could trap species in climate dead ends (see Chap. 5). But we might be able to help at least some of these species make a climate escape. The basic goal of assisted colonization is to help populations reach areas that we think will have suitable climate and other habitat requirements in the future.
One approach to doing that is to take climate change into account when we plan habitat reserve networks. This involves planning for and creating corridors of protected habitat that populations can follow as their ranges shift. We already use wildlife and habitat corridors as a tool for conservation, but when designing them, we have focused our efforts on helping to facilitate the current movement patterns of organisms as they negotiate existing barriers such as roads (see Figs. 8.24Figure 8.24.). An accessibility retrofit to an incidental wildlife crossing. (A) This culvert runs underneath State Route 118, a two-lane highway in Ventura County, California. It provides safe passage but is largely inaccessible to many animals, particularly smaller ones. Ramps have been installed to improve accessibility. (B) This black bear (Ursus americanus) probably doesn’t need the ramp, but it seems to appreciate it. Source: US National Park Service, https://flic.kr/p/2kSMhSA; b: https://flic.kr/p/2kSKD6Z. and 8.23
Figure 8.23. Trade-off in the ecosystem services that people in the United Kingdom want from former industrial sites (brownfields). A survey asked people to rate their preference for 28 distinct functions categorized into five broad categories. (A) A correlation matrix of the preferences. Some functions were frequently preferred together (positively correlated, indicated by blue circles), while others were rarely preferred together (negatively correlated, indicated by red circles). (B) The preference data presented as a cluster analysis. Respondents fell into one of two distinct groups. One group preferred services related to nature conservation and education, while another group preferred services related to development, food production, and recreation. Source: modified from Washbourne et al. (2020). ).
We are beginning to think about how organisms will negotiate future landscapes as they try to move in response to climate change. The pathways that organisms could potentially follow are called climate corridors. As I describe in Chap. 5, planning for climate corridors is challenging partly because the corridors aren’t simply networks of existing habitat. Instead, climate corridors will progressively develop as the microclimates that make up a landscape change in response to global climate. Climate corridors are paths of least climate resistance that organisms are likely to flow through as they get pushed away from deteriorating climate in one place and pulled toward improving climate in another. Where those paths of least resistance form and where they lead depend on the details of local geography (see Figure 5.16Figure 5.16. (A) Map of potential climate corridors across North America. This map predicts the expected traffic pattern of dispersing organisms as they move from locations with currently (1981–2010) suitable climate to locations predicted to have suitable climate in the future (2071–2100) under representative concentration pathway (RCP) 8.5. The red end of the scale indicates areas with expected high-traffic flow (i.e., climate corridors), while the blue end of the scale indicates areas with expected low-traffic flow. The map is based on the assumption that organisms will most likely move through areas that have the most suitable habitat at any given time. Areas with suboptimal or inhospitable habitat will act as barriers to movement. Mountains can form highly trafficked climate corridors because they are often the nearest source of suitable habitat in a changing climate. North-south-oriented mountains will form interconnected networks of suitable habitat through (B) passes and (D) ridgelines that will facilitate long-distance movement across the continent. In other cases, mountains will act as dead-end cul-de-sacs, isolating organisms on shrinking climate refugia surrounded by large expanses of unsuitable habitat (the gray shaded areas in C; note that region is the Madrean Sky Island archipelago). Source: modified from Carroll et al. (2018).). As global climate change progresses, some parts of a landscape will develop sharp contrasting differences in local climate, or the spatial extent of unique climates will shrink dramatically. For example, alpine climate zones in the low to middle latitudes are expected to shrink as global climate warms. For many alpine organisms, the only way off their shrinking climate mountaintop in search of more suitable habitat poleward will be to travel through increasingly inhospitable lowlands—a journey that most won’t be able to make. But in some parts of landscapes, physical features will create more gradual climate differences between adjacent habitat patches, or they will form climate refugia where the local climate will change relatively slowly compared to the surrounding region (see Figure 5.15
Figure 5.15. The Madrean ecoregion consists of an archipelago of sky island mountains amid a sea of lowland desert. The sky islands are climate refugia that support habitat types such as pine-oak woodland that used to be much more widespread during the Pleistocene. The photo is taken from the Chiricahua Mountains looking toward the smaller Dragoon Mountains in the distance. Sources: map modified from Villarreal et al. (2019); photo, US National Park Service, https://www.nps.gov/chir/learn/nature/sky-islands.htm.). For instance, high mountain passes could provide relatively cool pathways that lowland and even some high-elevation species could travel through in order to get to more climactically suitable poleward habitat. These parts of landscapes act as a sort of “slow lane” for pokey populations whose dispersal and movement would otherwise be outpaced by the overall rate of regional climate change.41 In addition to the barriers and pathways generated by shifting climate, organisms face more mundane barriers to their movement, such as large water bodies as well as human-created obstacles such as agricultural and urban landscapes. A high mountain pass might be of little use as a climate corridor if it has a burgeoning city within it.
Ideally, we would identify, protect, and even help create suitable climate corridors across landscapes. But that is easier said than done. On the technical side, we need to understand the current environmental requirements of populations, anticipate what regions will meet those requirements under future climate, and predict how changing climate will interact with geography to block or funnel the flow of populations across the landscape. Doing all that for one species is a daunting task, let alone for all the species in a complex ecosystem. But we are beginning to get a rough idea of the future needs and challenges even with our currently incomplete information. One study attempted to identify the likely pathways that plants and animals would take across the contiguous United States as they tracked shifting climate.42 Instead of creating detailed ecological niche models for a lot of individual species, the authors instead tried to identify climate corridors in a more general way. Their basic approach was to assume that the most likely pathways would form along routes where the temperature gradient between adjacent spots on the landscape was modest. Extreme temperature gradients would probably pose a significant barrier for organisms trying to move. The authors identified these pathways of gradual temperature change using a climate prediction model (see Section 4.5) to create a detailed spatial map of the degree to which temperature would change across natural areas in the United States by the end of the century. They then used an index of the temperature difference between adjacent patches to map out the most climactically favorable paths from present locations to locations that will have similar climate in the future.
The authors also accounted for any barriers that might hinder movement along otherwise climatically favorable pathways—these were mostly human-created barriers such as agricultural and urban landscapes. The authors found that just 41% of US natural land has enough unfettered climate pathways to allow species to track their current climate as it shifts across the landscape. Most of the climate pathways are in the West; less than 2% of natural area in the eastern United States currently has climate escape routes.43 That mostly reflects the fact that more of the natural areas in the East are isolated islands surrounded by large swaths of inhospitable habitat in the form of farms and cities.
It is not just the pathways that our activities can block. Another study estimated that 13% of the potential destinations for climate-displaced populations have greater human use intensity than where the climate colonists will be coming from, suggesting that these climate destinations will not have the capacity to support all the climate colonists that they will receive.44
We can use information like this to protect prime climate corridors, plan where to restore habitat in order to create climate corridors, and improve conditions in areas that will likely receive climate colonists. But creating or restoring extensive new protected areas involves sociopolitical challenges. In North America, only 20% of the lands that have high value as climate corridors or refugia are currently under any sort of conservation protection.45 Ensuring that these lands continue to function as corridors and refugia will require broad social consensus for action and the active support of local communities. Some of the approaches that I describe for managing our agricultural and urban systems in Chapters 7 and 8 could help. For instance, we could develop economic incentives and technical tools to help farmers and ranchers simultaneously manage their land both as sources of economic prosperity and as climate pathways.
Assisted colonization can also involve the active translocation of populations outside of their current range. As I describe above, a potential benefit of active translocations can be to help conserve species that are threatened or endangered in their intrinsic range. The introduction of Aldabra giant tortoises to the Mascarene Islands is an example. While the main goal of those introductions was to restore ecological functions lost when the endemic Mascarene tortoises went extinct, the translocations also provide a conservation insurance policy for the Aldabra giant tortoises. Aldabra is a particularly low-lying atoll in the Indian Ocean that has periodically been submerged during periods of high sea level in the Pleistocene. Each period of high water caused the local tortoise populations to go extinct. Tortoises recolonized when the atoll reemerged during periods of low water, most likely by colonists from Madagascar. The current period of climate-change-induced sea level rise is again threatening the Aldabra tortoises, only this time there are no longer any Madagascar tortoises to recolonize: those went extinct when we colonized Madagascar about 2,300 years ago. Introducing Aldabra giant tortoises to other islands in the Indian Ocean, including Madagascar, is a way to help conserve the last remaining bits of giant tortoise genetic and ecological diversity.46
We will likely try to actively translocate populations more frequently as the effects of climate change become more pronounced. Getting translocations to work is tricky, however. One of the basic technical issues in doing translocations is that we need to move organisms while climate conditions are still viable in the old range but are also tolerable in the new range. Take, for example, the tropical dry forests of Mexico. Tropical dry forest is abundant in the low to middle elevations of Mexico’s Sierra Madre Occidental, but the region is rapidly warming and drying. That is shifting the climate envelope required by many of its species up in elevation. But many of the endemic tree species such as Hesperalbizia occidentalis have poor dispersal ability. Dispersal is further hindered by extensive deforestation and habitat fragmentation that has occurred in the region. Assisted translocation is being explored as a way to help ensure that populations are able to establish at higher-elevation locations as climate shifts. A small-scale experiment conducted in Michoacán showed that translocated populations of H. occidentalis could survive, but only at elevations that are just slightly higher than their current elevation range. Elevations more than about 400 m above the current range are still too cold,47 suggesting that any translocation program would have to be a sustained and long-term project.
Some species are more amenable to translocation than others. For example, the adults of long-lived perennial plants can often tolerate a much wider range of environmental conditions than those that are required for germination or the establishment of juveniles. Sometimes adults can tolerate deteriorating climate in the home range even though conditions currently prevent the establishment of new individuals into the population. Such tolerance can buy us time to collect germplasm from the adults—such as in the form of seeds—that we can put into storage while we wait for conditions to become suitable for translocation someplace else. Similarly, conditions in the potential translocation range could still be unfavorable for recruitment while the adults are able to manage just fine. We might be able to pamper seedlings—such as providing irrigation or protection from frosts—just enough so that they are able to establish a viable population of adults. The adults could then persist until climate had changed enough to allow recruitment without our help.
Caveats and Concerns
Well-designed climate corridors, climate-change-resilient reserve networks, and active translocations are—at best—a last-ditch emergency response. They won’t help use avoid significant biodiversity loss if we keep following the worst-case carbon emission trajectories. For instance, while we can help some Mexican tropical dry forest species move upslope, the pine-oak forests that currently exist at higher elevations have no place to move to.48 It will be far better for biodiversity and us if we avert catastrophic climate change in the first place.
Introductions and population translocations have a number of other technical and conceptual issues that we need to work through. Right now, we have far more questions than answers. These fall into two broad categories.
How Do We Mitigate the Potential Risks of Introducing Populations?
Moving species into areas where they do not have an ecological or evolutionary history could create some of the problems that are associated with invasive populations (see Section 6.5). Also, managing translocations involves philosophical and conceptual questions similar to those that have arisen with our management of invasive populations (see Section 9.3). What happens if some of our translocated species contribute to declines of the existing local biodiversity? Should local human communities have a say in which are introduced to their local ecosystems? What if conflicts arise between people that want to translocate a culturally important species to a new area and those that don’t want a non-native species to be introduced to their local ecosystem?
How Do We Prioritize Which Species to Move?
Introducing or moving populations is costly and technically challenging in terms of rearing populations, keeping individuals alive during transportation, and establishing viable populations at the introduction site. It is likely that we will only be able to introduce or translocate a small number of populations that make up a tiny subset of all the species in ecosystems. How do we choose which species to put on the climate ark? Some criteria for prioritization include species that provide particularly important ecological functions (such as in the case of the Aldabra giant tortoise), species that provide important human services such as food production, species that we value for their cultural or historical significance, species that are the least likely to be able to adjust to climate shifts on their own, and species that are most amenable to the translocation process or that are most likely to be successful in the translocated range.
10.3 Assisted Evolution
Section 10.3: Assisted Evolution
In addition to moving populations to where we think conditions will be more suitable for them, we can attempt to influence the processes that drive evolution in order to help populations adapt to changing conditions. In other words, we can assist evolution. The term reflects a fundamental unease that many have with the concept. It tries to make a narrow distinction between the manipulative approaches we have used to develop domesticated animals and plants from approaches whose goals include biodiversity conservation. While some assisted evolution approaches do just nudge ostensibly natural evolutionary processes, others involve the more active design and engineering of evolutionary trajectories. Our ability to implement and even to consider these approaches has been made possible by the ongoing revolution in biotechnology.
Captive Breeding and Genetic Rescue
Some tools that fall under the broad umbrella of assisted evolution have been used in conservation biology since the early days of the discipline. Examples come from efforts to conserve critically endangered species from extinction. As species near extinction, captive breeding programs (sometimes called conservation breeding) are often used in a last-ditch effort to save the species. The loss of genetic diversity is a significant challenge that captive breeding programs must deal with. Critically endangered species experience severe genetic bottlenecks that dramatically reduce overall genetic diversity. With few breeding individuals to choose among, populations also become increasingly inbred. Both of those trends can rapidly accelerate if individuals are brought into captive breeding programs.
Captive breeding programs actively attempt to rescue captive populations from inbreeding and the loss of genetic diversity. One approach is to use pedigrees (similar to stud books in domestic breeding) or genomic data to maximize the genetic distance between mating individuals.49 That is often the best that we can do in situations where there are only a few individuals left in the wild. But often there is still some amount of population level genetic diversity left in the wild. In these cases, maximizing the genetic distance of captive mated individuals would tend to homogenize that remaining diversity. Instead, captive breeding programs try to maintain distinct breeding lines that correspond to the genetically unique wild populations. The distinct lines partly serve as an archive of the species’ remaining genetic diversity. Strategic mating across lines can be made in a delicate balance between maintaining genetic distinctness and causing inbreeding.
We can also genetically rescue wild populations that have experienced a genetic bottleneck or that are suffering from inbreeding. The main approach used for wild populations is planned introductions and translocations. A well-known example of genetic rescue in the wild comes from conservation efforts for the Florida panther (Puma concolor couguar), an isolated population of the North American subspecies of cougar—the same species that are called mountain lions in Southern California (see Fig. 8.6Figure 8.6. Mountain lion (Puma concolor couguar) P-35 in the Santa Susana Mountains, California. The US National Park Service has organized a monitoring program that includes a network of camera traps and GPS trackers—one is hanging from this female’s neck. The program is giving scientists a better picture of how the animals negotiate their urbanized landscape. It has also helped to make individuals local celebrities. Source: US National Park Service, https://www.nps.gov/samo/learn/nature/pumapage.htm.). In the 1990s, Florida panthers were on the verge of extinction with only about 25 remaining in the wild, and that population suffered from the effects of severe inbreeding, including physical deformities and poor breeding success. Individuals from Texas populations were translocated to Florida, which increased the genetic diversity and fitness of the Florida populations.50 While these cougar translocations were largely done without the help of detailed genomic information, going forward, we will be able to use genomic information to plan introductions that maximize the fitness benefit for the recipient population. For instance, we can screen potential source individuals for the presence of deleterious recessive alleles that might cause problems if they were introduced into inbred recipient populations.
The most extreme form of genetic rescue is resurrecting a species that has already gone extinct. In Section 10.1, I describe efforts to do that using reproductive cloning. That approach requires viable tissue (really a viable nucleus) from the extinct species, so it could only ever be used to resurrect a species that went extinct relatively recently or that was still represented by a forlorn individual or two—much to the chagrin of anyone with the dream of opening a Jurassic Park. A different approach has kept the hope of resurrecting decidedly extinct species alive. It involves editing the genome of a living species until it looks (more or less) like the genome of an extinct relative. The idea is sort of like starting with the text of H. G. Wells’s The Time Machine, changing the setting of the story, adding a few characters and plot lines, developing some new themes, and ending up with The Island of Doctor Moreau. As outlandish as that sounds, researchers at an organization called Revive and Restore have said they want to use this approach to resurrect extinct species.51 One of their ideas involves the passenger pigeon (Ectopistes migratorius), the last of which died in 1914 (Fig. 10.7). The plan starts with reconstructing the passenger pigeon genome from preserved specimens. This is not easy since the old DNA in those specimens has degraded, creating lots of fragmented pieces as well as gaps and errors. With enough specimens to guide us (and with some powerful computers and nifty software), we can stitch the pieces back together and attempt to fix the errors. The next step is to compare the reconstructed passenger pigeon genome with that of a related (and still alive) species such as the band-tailed pigeon (Patagioenas fasciata). After identifying where the two genomes differ, we could then use CRISPR-Cas9 gene editing to revise the genomes of band-tailed pigeon germ cells so that they resembled that of the passenger pigeon—in essence turning them into passenger pigeon germ cells. Those could then be implanted in a surrogate that would, in concept, give birth to the first passenger pigeon the world has seen in more than 100 years. Similar approaches have been proposed for other long-extinct species such as mammoths (see Additional Resources).
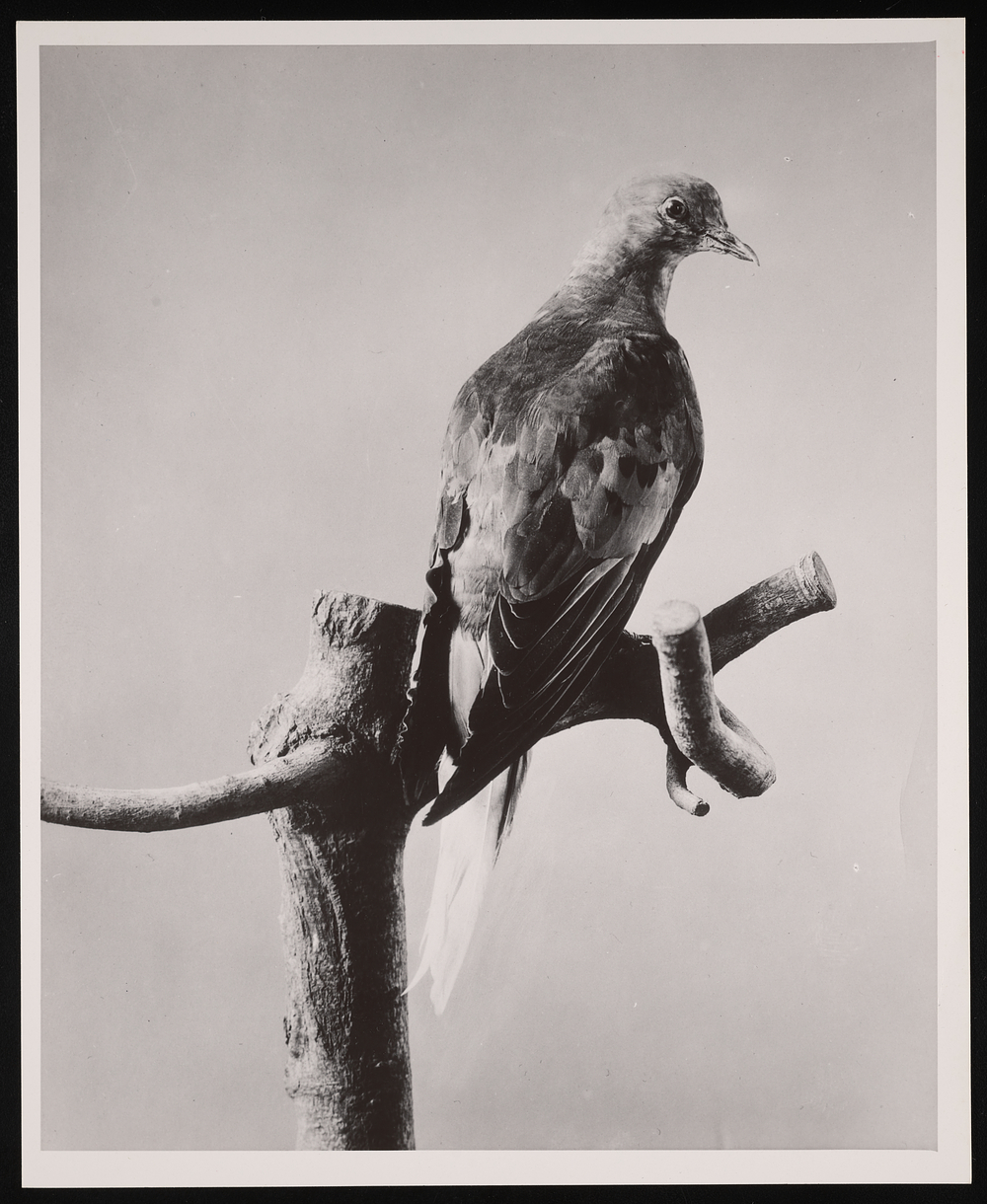
This concept is highly speculative, and there are a number of technical hurdles that are even bigger than the ones that have prevented reproductive cloning from becoming a practical tool. There are also significant ethical questions to reviving extinct species.52 One fundamental one is whether we can really call what we create a passenger pigeon or a mammoth. Our creations would have hybrid genomes that were at best a composite best guess of what the original genome of the species looked like. Even if it was more or less accurate, that genome would reflect a tiny fraction of the genetic diversity and none of the epigenetic diversity that once existed. The individuals would be islands unto themselves, divorced from the ecological connections and social memories of their ancestors, and born into a profoundly changed world. That pain of isolation would be particularly acute for highly social species such as the passenger pigeon and mammoth.
Helping Natural Selection
A fundamental way in which populations adjust to environmental change is through evolution by natural selection, which requires at least two things:
- Genetic variability that creates traits that are beneficial under some conditions and,
- Time enough for those traits to spread through populations.
Evolutionary change can happen remarkably quickly, even by our frenetic human timescales. I gave some examples of populations rapidly evolving to climate change in Chapter 5 and to conditions in our urban and agricultural environments in Chapters 7 and 8. Quick and easy evolution is certainly not universal, however. Factors just as often act to slow evolution down or constrain the paths it can take. These include low levels of genetic diversity within populations, the lack of unique traits that are beneficial under rapidly changing conditions, and behavioral or physical constraints that slow the spread of beneficial traits among the populations of a species. These types of issues will limit the capacity of many species to evolutionarily keep pace with the accelerating pace of change during the Anthropocene.
Reef-building coral species rank high on the list of concern. A range of Anthropocene changes are stressing corals, the two biggest being ocean warming and acidification (see Section 5.3). Corals have the potential to adapt to these changes. Some species are inherently more tolerant of warm temperatures or reduced alkalinity than others, and some species have a fair amount of intraspecific variation in physiological traits related to temperature and pH regulation. Despite that latent adaptive capacity, various constraints make it far from certain that coral species and populations will be able to adapt quickly enough, given how rapidly ocean conditions are changing. One constraint is that potentially useful alleles are not distributed evenly across populations and space. A genetic variation that bestows heat tolerance, for instance, might only exist in one population that makes up a small fraction of the geographic range of a species. Given how patchy and isolated reef populations are, it can take a long time for the beneficial allele to spread widely. Complicating matters is the fact that there are other Anthropocene conditions that corals need to adapt to, such as pollution and increased competition from free-living algae (see Section 6.8). Populations will need to acquire adaptive genes for those stresses along with those for heat and pH stress. Some populations and species might be able to overcome these obstacles quickly enough, but many more will not. The result will be coral reef systems that look and function very differently from today’s reefs if we continue our current trajectory of greenhouse gas emissions.53
But we might be able to lower some of the barriers to change by augmenting and manipulating the genetic diversity present within coral reefs. Historically, coral reef restoration has followed the general ecological restoration design principle of emulating the genetic and species composition that previously existed at a degraded location or that still exists at nearby locations (see Section 9.2). A typical reef restoration project involves collecting coral fragments from a degraded reef or from nearby reference sites, propagating the fragments asexually in a nursery (Fig. 10.8), and then transplanting the young coral back into degraded reefs to help jumpstart recovery.
<Fig. 10.8 here>
Coral reef managers are now considering ways to maximize the ability of restored populations to adapt evolutionarily to rapidly changing conditions.54 One way to do this is to make the restored populations as genetically diverse as possible, even if the result doesn’t look like what existed at the site previously. The idea is to provide a lot of variation for natural selection to choose from. For instance, instead of using nearby local populations as the sources of restoration transplants, we could use distant source populations that experience a range of different environmental conditions.55 Another approach might be to use transplants derived from sexual reproduction instead of asexual propagation. That is easier said than done, as coral species have unique (and often complex) modes of sexual reproduction that are dependent on subtle environmental conditions that we don’t understand very well. We are beginning to develop a better understanding of coral reproductive requirements (at least for some species), however, and we are beginning to successfully mimic the needed conditions under controlled environments.56 If we could reliably manipulate coral reproduction, we could also exploit a unique aspect of some coral species: their ability to naturally form genetic chimeras. Free-floating coral larvae tend to settle on reefs in close groups as they begin the sessile portion of their lives. Occasionally, genetically distinct individuals fuse to form a chimera. These chimeras tend to have enhanced traits such as faster growth, earlier sexual maturity, and a broader tolerance of environmental conditions.57 We could intentionally propagate chimeras for use in restoration.
Instead of just creating high levels of genetic diversity, we can also more specifically design the genetic mix of populations so that they contain useful traits. For example, reefs in the Kimberley region in northwestern Australia experience extreme high temperature, and their coral populations have evolved high levels of heat tolerance. These populations could be used as the source pool for reef restorations in other regions that currently experience more moderate temperatures but that are moving toward the temperature regime of the Kimberley reefs.58 Researchers have used functional genomics to identify genetic markers in some coral species that are potentially associated with heat stress tolerance.59 These types of markers would be handy screening tools to quickly identify individuals and populations that possess advantageous traits for use in restoration. We can also monitor how the process of natural selection is progressing on reefs and select the best performing genotypes for replanting or for use at another restoration site.
That type of husbandry makes ecological restoration similar to traditional agroforestry approaches where we give desirable species and variants a helping hand. You probably see the logical progression to that. If we can remove some barriers and help nudge natural selection along, why not intervene more directly?
Selective Breeding
In many ways, restoration ecology developed as an explicit rejection of approaches such as selective breeding used to develop our domesticated ecosystems (see Section 9.2). Selective breeding is now being considered as a tool for biodiversity conservation. We even have a few working examples. Several come from efforts to develop disease tolerance in populations that have been severely affected by the introduction of non-native pathogens. One of these is the effort to save the American chestnut (Castanea dentata) from extinction. American chestnut used to be an iconic, functionally important tree of the eastern deciduous forest of North America, but populations declined dramatically after chestnut blight fungus (Cryphonectria parasitica) was introduced from Asia in the early 1900s (Fig. 10.9). Today, the species hangs on the cliff edge of extinction, with just a few surviving adult trees mostly planted in urban landscapes of the relatively blight-free western United States. In the 1980s, the American Chestnut Foundation started a breeding program with the goal of developing blight-tolerant American chestnuts. They started by crossing American chestnuts with the blight-tolerant Chinese chestnut (Castanea mollissima). A big downside to this approach is that the crosses produce hybrids that are a kaleidoscopic combination of American and Chinese chestnut traits. What we really want is an American chestnut with one new trait: blight resistance. Getting there involves a laborious process of screening the resulting hybrids for blight resistance and then backcrossing promising ones with pure forms of American chestnut to produce hybrid forms that are blight resistant but that retain most of the other characteristics of American chestnuts. By 2020, the efforts of the American Chestnut Foundation had produced some promising candidates, and field trials had begun.60
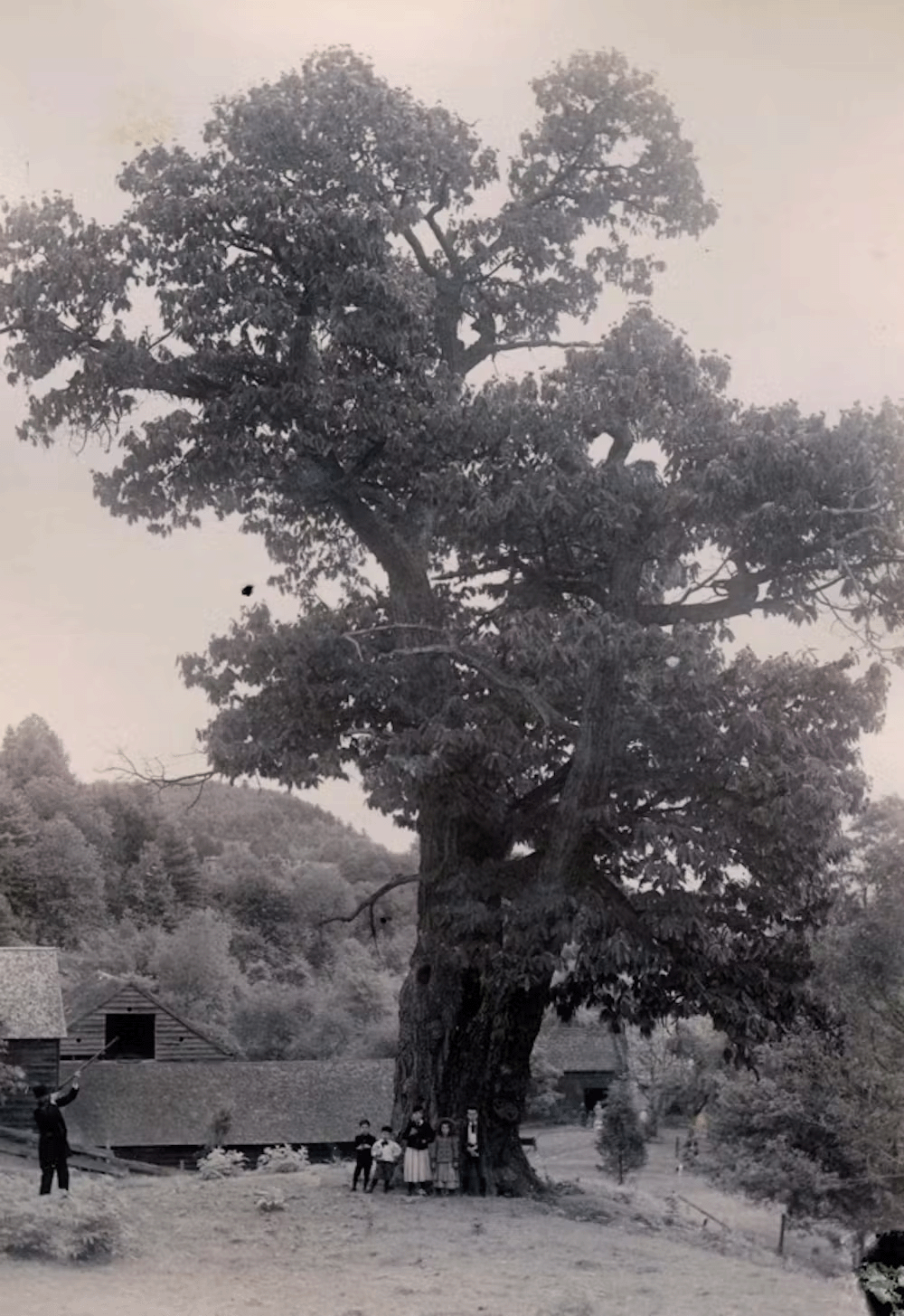
Genomic tools can be used to help speed up the selective breeding process. For example, we are beginning to identify coral genes that play roles in stress mitigation, and we are developing genetic markers that are associated with traits such as heat tolerance. We can use marker-assisted selection to plan crosses and to quickly screen the resulting offspring for desirable traits without having to do the time-consuming process of growing up the corals and exposing them to stressful conditions. Some early trials suggest the promise of the approach. When researchers crossed colonies of the staghorn coral Acropora spathulata from the warm northern end of the Great Barrier Reef with colonies from the cooler southern end, the resulting hybrids were 13 to 26 times more heat tolerant than the cool-adapted southern parents.61
Selective breeding has several drawbacks from the standpoint of biodiversity conservation—these were among the reasons why early restoration ecologists rejected selective breeding as a tool in the first place. One fundamental one is that breeding involves strong selection that tends to significantly reduce the original genetic diversity present in wild populations. Some of the variation lost during breeding likely involves important adaptive traits that help populations in their unique local environments. That is one of the reasons why many domesticated plants and animals tend to do poorly outside of the domesticated ecosystems we design for them. A related issue is that breeding creates hybrid genomes that merge two or more distinct genetic lines. That merger not only homogenizes genetic information, but it also creates novel combinations. Some of that novelty is desirable and is even the goal of making the crosses. But we don’t often understand what the effects of all the resulting novel combinations will be. This is particularly so if our goal is to introduce the hybrids into natural ecosystems. For instance, about 17% of the blight-tolerant American chestnut hybrid genome comes from the Chinese chestnut.62 It is far from clear what functions the missing parts of the American chestnut genome play or how their absence will influence the ecology of the hybrids once they are transplanted into wild populations. We also don’t know what impact the 17% of the Chinese chestnut genome will have on wild populations or on the eastern deciduous forest ecosystem.
There are some approaches to mitigate these issues in selective breeding. One, as was done in the chestnut breeding program, is to use backcrosses with wild-type parents in an effort to retain as much of their original characteristics as possible. The chestnut breeding involves creating interspecific hybrids, but the backcross approach can also be used in cases were breeding simply involves intraspecific crosses. This could allow us to retain the unique ecotype traits of local wild populations even in selectively bred forms. Another approach for retaining wild genetic diversity in a breeding program is to start with genetically distinct wild source populations and keep the genetic lines separate during the breeding process. The hope is that doing so will produce different breeding lines that in aggregate represent the full range of genetic diversity present in wild populations even though each individual line differs a bit from their respective parent populations. That is the approach the researchers who bred the heat-tolerant staghorn coral used. In aggregate, their different breeding lines retained much of the original population genetic variation even after several generations of selection.63
Genetic Engineering
Genetic engineering provides another set of tools that could be used to develop Anthropocene-adapted populations. Some genetic engineering techniques enable relively precise genomic manipulations, which can mitigate some of the undesirable effects of selective breeding. The effort to develop blight-resistant American chestnuts provides an example. A different group of researchers from the ones doing the selective breeding have successfully created blight-tolerant forms of American chestnut using transgenic techniques to insert a gene from wheat. The gene codes for an enzyme that breaks down oxalate, which is produced by the pathogen and forms the cankers that eventually kill trees. The transgenic chestnuts can achieve blight tolerance that is comparable to that of Chinese chestnut and much greater than that exhibited by the American chestnut-Chinese chestnut hybrids. Also, with the exception of the single insertion of the oxalate oxidase gene, the rest of the American chestnut genome remains intact. Because the tolerance trait is inherited like a dominant allele, in concept, the transgenic trees could be used as mother trees to spread the resistance trait through wild populations, thus preserving the remaining genetic variation and local adaptations that exist in the remnant populations of American chestnut.64 In contrast, several different genes control the blight resistance of the hybrids. This creates a complex pattern of polygenic inheritance that is difficult to predict. It is far less clear how or even if that resistance will spread through remaining wild populations.65
We could also use genome editing approaches such as CRISPR/Cas9 to insert an advantageous gene or to switch on or silence gene expression pathways. In 2018, researchers reported successfully using the CRISPR/Cas9 system to silence three genes in a species of staghorn coral Acropora millepora.66 The researchers in that study used CRISPR/Cas9 to help them understand the functions that coral genes code for: a great way to see what a gene does is to stop it working. But it is easy to see how we could use CRISPR/Cas9 to design new forms of coral that had a range of desirable traits.
A significant constraint to using selectively bred or bioengineered individuals for conservation is ironically related to the happy fact that the populations of most species are still large. Coral reefs are a good illustration. Although they have suffered severe declines and they are facing significant threats, coral reefs still span the tropical waters of the world. We can potentially use climate-designed coral to help restore degraded reefs here and there, or perhaps to establish a few new populations along the edges of existing ranges. But those applications are small in scale compared to the vast global distribution of coral reefs. Plus, reef restoration happens after the existing coral communities have suffered severe degradation. We might be able to restore a few of the coral species that were lost, but we are far less able to restore the entire biodiversity of the complex reef system once it has been lost. How do we overcome the significant logistical challenge of upgrading a global ecosystem? Can we upgrade coral proactively so that the reef systems they support don’t suffer declines in the first place?
As with transgenic blight-resistant American chestnuts, we could seed existing reefs with a relatively few individuals that have been bred for climate-adapted traits. In concept, those advantageous traits would spread through the population via natural selection. But we can only practically introduce a relatively small number of individuals, making their climate-adapted genes rare in populations. In addition, the normal pattern of inheritance sets a constraint on how fast those genes could increase their frequency, even if they are beneficial (Fig. 10.3). In addition, although heat stress events are becoming more frequent,67 they are still a periodic phenomenon. As a result, the selective advantage for an individual with a climate favorable gene may only come about every few years or so. Those factors would make any traits we breed or engineer susceptible to being lost from populations because of chance and potentially being slow to propagate through populations. Gene drives could provide a way around these constraints. In one optimistic scenario, we could use CRISPR/Cas9 to create climate-edited genomes for a diverse range of functionally important coral species, then use a gene drive system to propagate the advantageous genes through wild populations. Of course, there are substantial concerns over the use of gene drives, even if we are using them to spread presumably beneficial genes as opposed to deleterious ones.
Acclimatize Individuals and Populations
Some forms of acclimatization aren’t particularly amenable as conservation tools because they produce transient, quickly reversible changes in individuals. The forms of acclimatization linked to epigenetic mechanisms have much more promise. Once again, coral conservationists have been at the vanguard of developing potential approaches. Many coral species are particularly adept at acclimatizing to a range of stresses such as high temperature, and it seems likely that at least some of that ability is related to epigenetic mechanisms.68 This opens the possibility that we can actively acclimatize coral populations ourselves and that some of the acquired traits could be passed down to future coral generations. One idea is to use coral nurseries (Fig. 10.8) to acclimatize populations to stresses such as high temperature or reduced alkalinity. To do that, we could locate the nurseries in areas that experience stressful conditions or perhaps manipulate physical conditions in the field or in controlled laboratory settings. We could then transplant the acclimatized individuals at restoration sites. Even though conditions at the restoration sites might be relatively benign, the transplants would hopefully retain epigenetic changes in their genomes that would allow them to persist if conditions deteriorated. This is the converse process to hardening off plants grown in a greenhouse before transplanting them into more stressful field conditions.
Some of the ability that corals have to tolerate and adjust to stressful conditions is mediated through their microbiomes. This includes the obligate symbiosis that reef-building corals form with dinoflagellates in the genus Symbiodinium, as well as a range of other microorganisms that play important roles, such as helping in nutrient acquisition and disease resistance.69 An approach to acclimatizing corals could be to design probiotic cocktails that inoculate corals against stressful conditions. For instance, there are many different genetic types of Symbiodinium that vary in their thermal tolerances. Young coral inoculated with warm-adapted Symbiodinium grow well in high temperatures, while coral inoculated with cooler-adapted Symbiodinium do poorly in heat.70 A handy aspect of Symbiodinium is that they can live free outside of coral bodies, allowing us to readily culture them in the lab. We could therefore raise naturally occurring beneficial strains of Symbiodinium and other probiotics, much like we do Greek yogurt or kombucha. We could also use the lab cultures as rapid evolution machines. In one experiment, researchers exposed cultured Symbiodinium strains to steadily increasing temperature over about a year (about 50 generations). At the end of the year, the heat-selected populations had evolved greater heat tolerance relative to the original wild type strains.71 We could also develop beneficial forms of Symbiodinium using selective breeding or bioengineering techniques.72
Caveats and Concerns
Many of the approaches I have outlined in this section are currently more creative ideas than practical solutions. Just as with assisted colonization, there are a number of technical and conceptual hurdles that need to be overcome before many assisted evolution ideas become working tools. One overarching hurdle is simply the enormous scale at which we would need to apply the tools. Spatial extent is one aspect of that, but perhaps not the most significant. As the rest of this book outlines, we have already transformed large swaths of the planet. We should be just as adept and spreading heat-tolerant coral or disease-resistant trees around the world as we have spread the species composing our domesticated ecosystems. Far more daunting is trying to guide the evolutionary trajectories of all of Earth’s biodiversity. We face enormous challenges just trying to breed climate-adapted varieties of the few hundred species we use as food crops. Those challenges are trivial compared to trying to do the same for all of Earth’s biodiversity. We will undoubtedly need to prioritize which species and aspects of biodiversity we attempt to evolutionarily assist. But it is not at all clear what the best approach to that prioritization should be. Should we prioritize functionally important species such as large reef-forming corals and dominant forest tress? Or should we focus on those species that are least likely to be able to adapt on their own either because of their unique biology or because we have created limiting conditions that constrain their evolution?
Another broad technical challenge is how to prevent our solutions from becoming problems. Even more so than with assisted colonization, creating and then releasing novel forms of organisms into ecosystems could have unexpected consequences. Some technologies such as gene drives have more inherent risk than others. But the issue is more nuanced than obvious cases such as a rogue gene spreading its way through the biosphere. Approaches such as selective breeding and genetic engineering create organisms that are different from their unmanipulated relatives. It is difficult to predict how those differences will manifest themselves in the functioning of populations and ecosystems. It can also be difficult to decide whether any changes are problems. What if blight-resistant American chestnuts are a little less palatable to native insects than non-blight-resistant forms? Is that sufficient reason to not use them in a reforestation program?
That technical issue relates to one of the main philosophical concerns people have with assisted evolution. Are we really conserving Earth’s biodiversity by creating new forms of it? That question is part of our broader struggle with conceptualizing nature and our relationship with it (see Section 9.1). Many people worry that the distinction between nature and our domesticated ecosystems is becoming increasingly blurry. To them, conservation should strive to preserve the distinction, not contribute to the blurriness. A practical aspect of the philosophical concern is that we might put too much emphasis on technological solutions. Some people worry that biotechnology provides a siren call that lures us away from social and political solutions that would create a healthier working relationship between us and the biosphere. The technical hurdles to breeding climate-resilient coral reefs or disease-resistant forests can at times seem more tractable than finding global consensus on limiting carbon emissions. But even the most optimistic futurists admit that biotechnology by itself only offers us the chance to save a portion of Earth’s existing biodiversity.
10.4 Social Engineering
Section 10.4: Social Engineering
Organisms are not simply reflections of their DNA. They communicate with each other, they learn from each other, and they develop rich cultures. This social life allows populations to transcend the physical constraints of anatomy, physiology, and time. Our own society—and the Anthropocene it created—is the prime example. But all organisms have a social life that helps them negotiate the vicissitudes of the world and adapt to changing conditions and unique environments. We largely ignore the social lives of other organisms. Self-absorbed in our own social existence, it hardly crosses our minds that other organisms have one as well. If it does, we usually only consider organisms that act most like us (e.g., our primate relatives) or organisms that we co-opt into our own lives (e.g., our pets). Even in these more familiar cases, our ability to understand and interact with other organisms is stymied by a vast gulf of misunderstanding. As the philosopher Ludwig Wittgenstein put it, “If a lion could talk, we would not understand him.” Wittgenstein realized that communication barriers go far beyond the rudiments of language. The world of a lion is outside of our ability to understand using our words—and if a lion could use our words to describe its life, it wouldn’t be a lion anymore.
That philosophical divide has hampered our study of nonhuman societies. Most of what we know comes from a few groups of animals, and that knowledge has been filtered through our biased human-centric perspectives. We have been making slow progress in our understanding, however, and recent years have seen an explosion in research that explores nonhuman culture in innovative ways. This work suggests that culture is much more widespread, diverse, and important than we had appreciated. We are even beginning to suspect that plants might have just as rich social lives as animals, only in ways that are very different.73
We are also realizing that nonhuman culture is another aspect of biodiversity that is being radically disrupted and altered. That realization is driving us to develop strategies and approaches for conserving the cultural aspects of biodiversity. We are just beginning to explore ways of interacting with nonhuman societies that will help humans and nonhumans better weather the tumult of the Anthropocene.
The Social Component of Biodiversity
Much of our increased understanding of nonhuman culture has come from freeing ourselves from the biases and preconceptions shaped by our own culture—no easy thing to do! Until recently, we tended to look for signs of our culture in other organisms. Not surprisingly, we didn’t find much. Although it is perhaps remarkable how much of ourselves we can project on to other organisms. Most natural history films are organized as melodramas just as compelling as any reality TV show. We even managed to embroil a Celebes crested macaque (Macaca nigra) in a copyright battle over a selfie they took (Fig. 10.10). But we have become more cognizant of how restricting our human perspective can be, and behavioral scientists have begun to broaden their definition of culture.
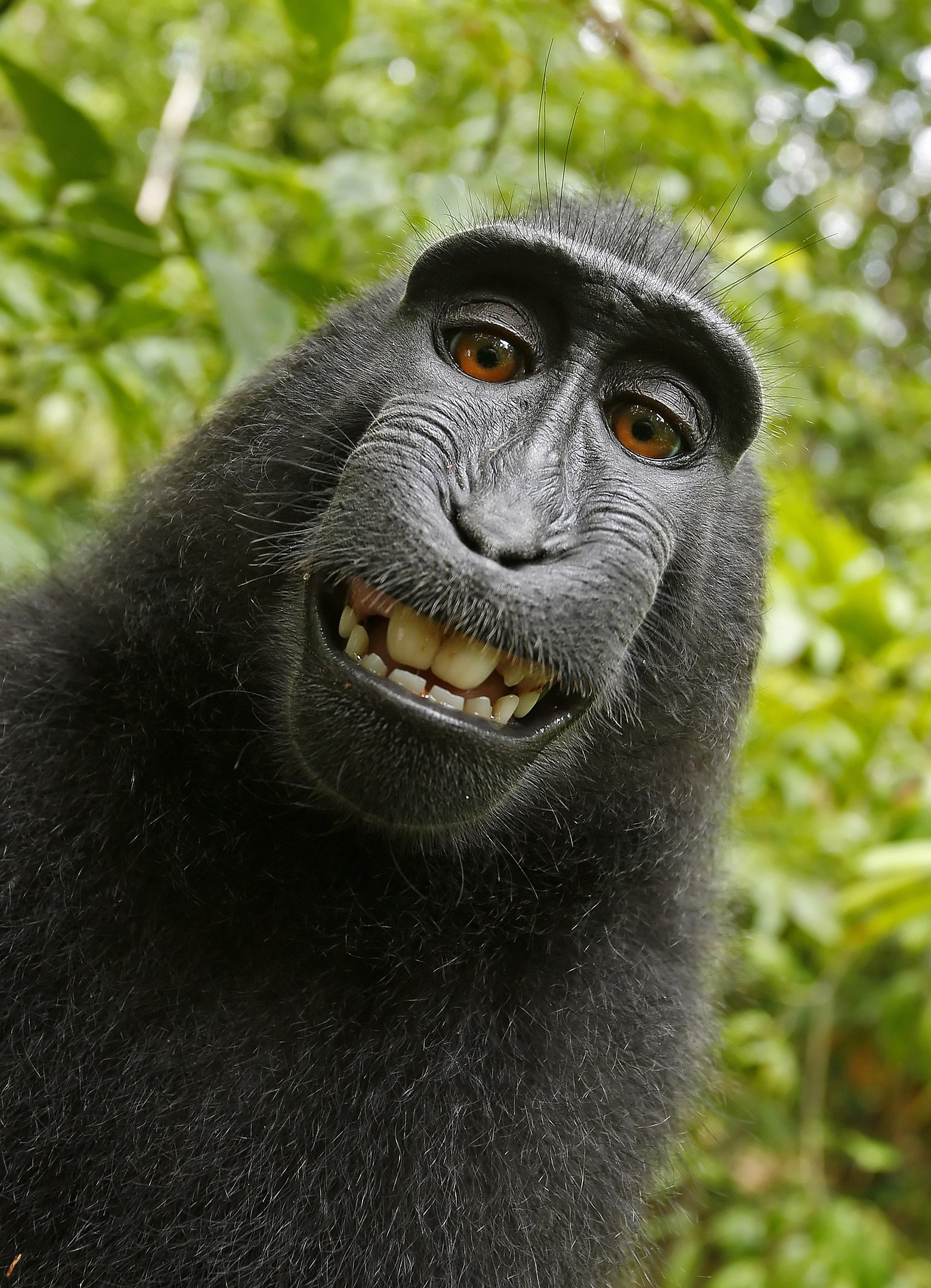
One way to get out of our human-centric respective is to think of culture in generically abstract terms. The current biological definition of culture is based on the process of social learning: knowledge and behaviors that are acquired by observing other individuals or being taught by them. Social learning contrasts with learning gained from personal experience or repetition. For instance, I have learned by dreary repetition the various routes I can take for my daily commute. But that type of learning by personal discovery is not nimble if conditions change. I am easily flummoxed if an accident or road construction blocks a familiar route. But I can use a smartphone app that allows me to tap information shared among a social network of other drivers. The collective power of many drivers observing conditions, looking for alternative routes, or remembering a time when something similar happened in the past can identify solutions much faster and more efficiently than any individual could do on their own.
Information exchange and collective community learning create a shared body of knowledge and shared behaviors that transcend the individual. These collective, socially learned traits are culture.74 The definition is often narrowed a bit to define culture as social learning that results in changes to populations that are sustained over time and that often get passed down through generations. Put more succinctly, learning creates traditions. The passing down of traditional knowledge to the next generation is particularly interesting because it is another way—in addition to genetic and epigenetic inheritance—that populations can adapt over time to changing conditions.
The more we look for social learning, the more it seems to be ubiquitous among animals (Table 10.1). The traditions that social learning creates can be characteristics that are just as distinguishing of populations as genetically based traits. For example, many animals have developed regional dialects in their calls and songs. There are many examples across a diverse range of taxa, but we have a particularly detailed understanding of the regional twangs in the songs of birds. If you live in North America, you probably have heard the sweet whistle of white-crowned sparrows (Zonotrichia leucophrys). Although the overall structure of the song remains distinctively white-crowned sparrow no matter where you hear it, the details of the song vary considerably from population to population, much like all the different versions of English you can hear across different parts of North America. Even populations that are just a few miles apart can have consistently distinctive songs. Check out the recordings of three different white-crowned sparrow populations around Marin County, California, in the Additional Resources.
Examples of Social Learning in nature | |
---|---|
Species | Social Learning |
Humpback whale (Megaptera novaeangliae) | Males learn the lyrics to popular local songs, which are passed down through generations. Sometimes new songs spread across regions like a viral video, although over years not days (Garland et al., 2011). |
Fruit fly (Drosophilia melanogaster) | Female flies choose mates based in part on watching the choices of other females. Preferences for random differences in male traits like color soon become the local cultural norm among females. The cultural traditions get passed down through generations (Danchin et al., 2018). |
Common octopus (Octopus vulgaris) | Octopuses can learn to do complex tasks by watching other octopuses. Other species with largely solitary lifestyles also do social learning, including other cephalopods such as cuttlefish (Sepia officinalis) and a number of fish species. This indicates that group living is not a prerequisite for culture (Fiorito and Scotto, 1992). |
House sparrow (Passer domesticus) | House sparrows have different personalities. Some are adventurous, while others are wary of new conditions. But the cautious learn from the daring which new things are safe and how best to negotiate them. Social adaptation may be one reason why house sparrows have successfully spread around the world (Kelly et al., 2020). |
African Elephant (Loxodonta spp.) | The oldest individuals in elephant societies are important repositories of generational social knowledge. We used to think that the female matriarchs of social groups did most of the teaching. But it seems that even older males, who typically are roaming loners, are important teachers and role models for young males (Allen et al., 2020) |
Examples of Social Learning in nature | |
---|---|
Species | Social Learning |
Humpback whale (Megaptera novaeangliae)![]() |
Males learn the lyrics to popular local songs, which are passed down through generations. Sometimes new songs spread across regions like a viral video, although over years not days (Garland et al., 2011) |
Fruit fly (Drosophilia melanogaster)![]() |
Female flies choose mates based in part on watching the choices of other females. Preferences for random differences in male traits like color soon become the local cultural norm among females. The cultural traditions get passed down through generations (Danchin et al., 2018). |
Common octopus (Octopus vulgaris)![]() |
Octopuses can learn to do complex tasks by watching other octopuses. Other species with largely solitary lifestyles also do social learning, including other cephalopods such as cuttlefish (Sepia officinalis) and a number of fish species. This indicates that group living is not a prerequisite for culture (Fiorito and Scotto, 1992). |
House sparrow (Passer domesticus)![]() |
House sparrows have different personalities. Some are adventurous, while others are wary of new conditions. But the cautious learn from the daring which new things are safe and how best to negotiate them. Social adaptation may be one reason why house sparrows have successfully spread around the world (Kelly et al., 2020). |
African Elephant (Loxodonta spp.)![]() |
The oldest individuals in elephant societies are important repositories of generational social knowledge. We used to think that the female matriarchs of social groups did most of the teaching. But it seems that even older males, who typically are roaming loners, are important teachers and role models for young males (Allen et al., 2020). |
Many of the cultural differences that arise among populations develop for seemingly random reasons. Human fashion fads are great examples (I deny ever owning a pair of ultrawide JNCO jeans). Social learning can reinforce and spread random cultural differences, similar to how natural selection can spread random mutations. The different white-crowned sparrow dialects probably arose at least in part by faddish accident. A particularly creative or forgetful bird altered a song that was then copied by others in the population. Learning feedbacks made the new song a population-wide characteristic. One difference between social learning and natural selection is that social learning can spread traits that don’t necessarily have any fitness benefit for organisms. In some cases, however, strong social pressure can turn what started out as seemingly innocuous random variation into traits that do influence fitness. An example comes from fruit flies. Female fruit flies choose mates based in part on watching other females choose their mates. In many cases, the initial choices made by females are random and based on traits like color that have no clear fitness benefit independent from the random female preference for it. Since females copy each other, that initial random choice can soon become the local cultural norm among females, and the cultural tradition gets passed down through generations. That creates a strong selective advantage for males that possess the culturally preferred traits over those that don’t.75
In other cases, social learning is more than just the process that spreads traits—it is a unique trait itself that can create fitness advantages for organisms. For instance, many animals have a rich cultural heritage related to finding and capturing food. This includes learned knowledge about where and when the best food is available as well as the best strategies for capturing it. Bottlenose dolphins (Tursiops aduncus) living in Shark Bay, Western Australia, learn the best foraging strategies from their mothers as well as from other more distantly related members of the population.76 The strategies include how to use tools such as sponges and empty mollusk shells to capture prey (see the videos in the Additional Resources).
An unresolved question in animal behavior is the degree to which the information, knowledge, and characteristics that are passed through social learning persist, accumulate, and incrementally evolve over generations like they do in human populations—or like how genetically based heritable traits accumulate in populations and evolve over time as a result of natural selection.77 The long-term persistence and adaptive transformation of knowledge are powerful forces. Our own long history of technological innovation is a prime example (see Chap. 1). We know that animals can retain and pass down information across many generations—the songs of humpback whales (Megaptera novaeangliae) are one example.78 We also have some evidence that passing down cultural knowledge through generations can enhance fitness. An example comes from reintroduced moose (Alces alces) and bighorn sheep (Ovis canadensis) populations in North America. Most moose and bighorn sheep populations (like many other herbivores) seasonally migrate as they follow the sequential availability of their forage across the landscape—a behavior that has been called “green-wave surfing”.79 Both moose and bighorn sheep have suffered population declines over the past couple hundred years, and conservation efforts have included reintroducing populations to areas where they had been extirpated.
Initially, the introduced populations did a poor job of migrating to track the spatial shifts in their food. This is understandable because the introduced individuals didn’t know what the local patterns were—much like you might not know where the good restaurants are after you first move to a new city. But the moose and bighorn sheep slowly figured things out, and this knowledge was passed down to subsequent generations. Interestingly, over the years, the reintroduced populations got increasingly adept at tracking food resources, suggesting that social knowledge was accumulating and improving over time.80 One fundamental difference between our culture and that of other animals is written language. Written language—along with all the associated storage and transmission tools such as libraries and the Internet—helps us retain and build upon cultural knowledge. In contrast, animal culture is exclusively stored in the knowledge of currently living individuals. That makes animal cultures particularly susceptible to changes in the individual composition of populations.
Culture and Conservation
We are beginning to better appreciate the fact that our activities are dramatically reshaping animal culture just as much as other aspects of biodiversity. Chimpanzees (Pan troglodytes) provide an example. Chimpanzees have a rich culture that varies among different chimpanzee populations. We have documented at least 31 different behaviors used by West African chimpanzees, including making and using tools to capture favorite foods such as termites and honey. But human activities disrupt chimpanzee societies and simplify their culture. Chimpanzees living in areas with a high degree of human disturbance have considerably less complex behavioral repertoires than chimpanzees living in areas with less human disturbance.81 We don’t know the exact reasons for the simplification, although we have some good guesses. For instance, poaching just doesn’t kill a few individuals; it also removes the cultural knowledge and expertise those individuals possessed—things such as how to make and use tools.
The loss of culture can negatively affect the fitness of populations and in some cases can help drive populations toward extinction. An example comes from the critically endangered regent honeyeater (Anthochaera phrygia). Regent honeyeaters are gregarious nomadic songbirds. Until recently, large flocks traveled hundreds of kilometers across southeastern Australia, but habitat loss has reduced their numbers to an estimated 200–400 sparsely scattered individuals. Male honeyeaters sing a song to attract mates and establish territories, which they learn from older males. Males don’t sing when they are rearing their offspring, so the young males have to learn regent honeyeater songs from birds who aren’t their fathers. Finding song tutors has become increasingly difficult as population numbers have plummeted. In the absence of species appropriate song tutors, many young males now often learn the songs of other species (see the video in Additional Resources). Even if young birds do manage to learn something that resembles a regent honeyeater song, it is often a truncated or otherwise atypical version. Those ersatz songs confuse and turn off females, significantly reducing the reproductive success of the males that sing them.82
Cultural disruptions also complicate efforts to conserve and restore populations. For example, efforts to conserve regent honeyeaters include a captive breeding program. But captive-bred males have clipped and abnormal songs, similar to those of lonely free-living males. On top of that, reintroduced populations are by practical necessity small, and they often lack the population density that would enable males to learn a standard regent honeyeater song instead of a poor cover of some other species’ song. The loss of cultural knowledge in captive-bred populations is widespread. Another example comes from African elephants (Loxodonta africana). Older elephants—particularly female matriarchs—are the repositories and teachers of the cultural knowledge that helps elephants negotiate their environment. Just one bit of that knowledge is how to expertly deal with threats from lions (Panthera leo).
Elephants are savvy when it comes to dealing with the threat. A lone lion is mildly concerning, but elephants don’t let it disrupt important activities like feeding. They will keep an alert eye on it but otherwise go about their day. A pack of lions is a much bigger threat. If elephants spot a pack of lions, they form a defensive huddle with a phalanx of big adults that protects a huddle of the group’s young and vulnerable individuals. This professionalism in the face of lions is culturally learned knowledge. Elephant populations that have been reestablished from young (usually unrelated) orphaned elephants lack that cultural skill. They freak out at the site of a single lion and immediately go into a full-on defensive huddle—much like I would probably freak out if I ever came face-to-face with a free-roaming lion (Fig. 10.11). In addition to having not learned the finer points of dealing with lions from their elders, it is possible that the orphaned lions have been psychologically scarred from the trauma of becoming and being an orphan.83
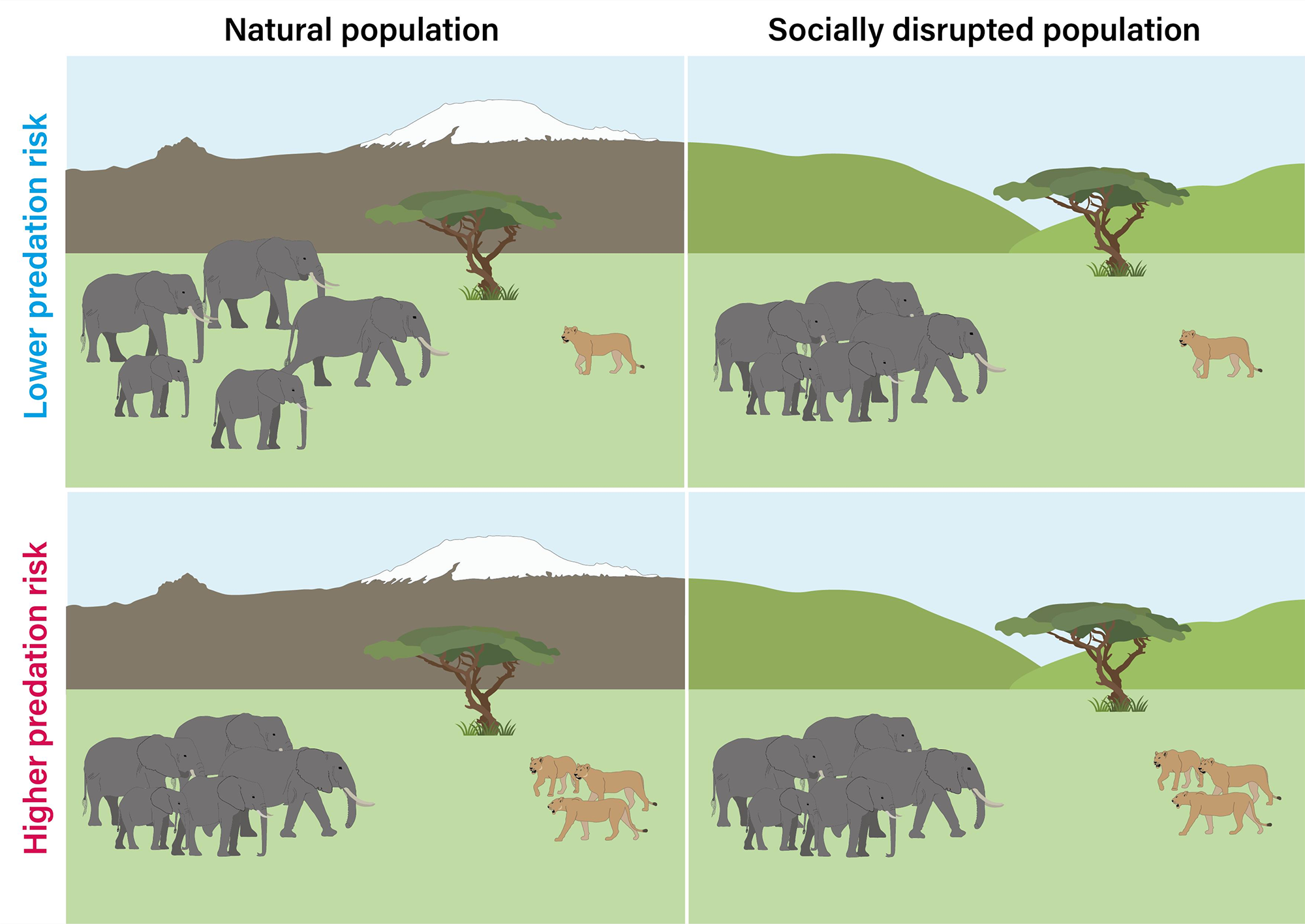
We are beginning to explicitly account for and make use of animal culture in our conservation efforts in four broad ways.
Using Cultural Traits to Help Prioritize Which Populations and Individuals to Conserve
The increasing scale of biodiversity loss is forcing us to triage our conservation efforts. One common prioritization is to conserve a representative sample of the existing biodiversity present in a species. This involves identifying populations that collectively reflect the spectrum of unique differences and variations that a species contains. We have typically used genetic composition or genetically based phenotypes as the metrics to describe population variation, mostly because we know how to measure those things. But we also know that genetically based variation plays an important role in helping populations—and collectively the species as a whole—adjust and adapt to changing conditions. In the parlance of conservation biology, these unique populations are called evolutionarily significant units. Much like a diversified financial portfolio can help hedge the risks of market crashes and economic downturns, a diverse genetic and functional trait portfolio can help a species hedge the risks of the Anthropocene. In addition to genetically based traits, cultural traits can be an important part of a well-balanced species portfolio.
In some cases, cultural differences reflect the same processes of geographic isolation and environmental variation that drive genetic variation and functional trait adaptation. We therefore can use cultural traits as indicators of broad suites of unique traits that a particular population possesses. For example, dolphins communicate in part by using a language based on whistles. Each dolphin species has its own language, and populations within a species have their own dialects—similar to the different song types of humpback whales (Table 10.1). A study that recorded whistles from many populations across several species of dolphin in the Atlantic Ocean and Mediterranean Sea found that the differences in whistle types broadly corresponded with other aspects of dolphin diversity, such as population genetic structure and differences in how populations adapted to local environmental conditions. Getting acoustic data on whistle variation is a bit easier than collecting genetic information or doing detailed studies on the ecology of each dolphin population. Whistle variation could therefore be a useful tool for ensuring that we conserve as much overall dolphin diversity as possible.84
In some cases, cultural variation could be an aspect of biodiversity that we want to conserve for its own sake. This could be because we admire and value the cultural variation or because we suspect that the cultural variation provides a fitness value to the population—or both. For example, the tool using populations of bottlenose dolphins and West African chimpanzees are part of the cultural identity of their respective species. If we want to preserve chimpanzees and bottlenose dolphins, we should make an effort to conserve that cultural identity. In addition, those unique populations are repositories of cultural knowledge that may help their respective species negotiate the turbulent changes of the Anthropocene. We are beginning to make conserving cultural differences an explicit priority. The authors of the study that found that our activities are eroding chimpanzee culture suggest establishing chimpanzee cultural heritage sites in order to protect culturally unique populations.85 The United Nations Convention on the Conservation of Migratory Species of Wild Animals calls for the protection of the behavioral diversity present in animal populations.86
The relationships among individuals in a society are organized into social networks, which have a few general characteristics. They tend to be subdivided into modular subgroups whose individuals interact more frequently with each other than with those in other subgroups. These groups reflect different—often nested—aspects of social life. For instance, western gorilla (Gorilla gorilla) society is organized around small family groups consisting of a single male, one to a few females, and their young children. Individuals in family groups also interact nonrandomly with individuals in other family groups that are analogous to groups of friends in human society. Young bachelor males also form their own social groups as they await the chance to form a family group.87 Another general characteristic of social networks is that some individuals tend to be more socially connected than others—sort of like social media influencers. For instance, elephant matriarchs play an outsized role in storing and transmitting cultural knowledge.
The details of these generalities vary considerably across species. We can map out the important social connections present in populations (Fig. 10.12) and use various statistics to describe the differences in quantitative ways—an approach known as social network analysis. Social network maps can help us to identify specific individuals, types of individuals, or groups that are particularly important for maintaining social cohesion, and we can design conservation efforts in ways that prioritize those important social actors. We can also adjust our management in ways that reduce the risk of causing social disruption. For example, commercial fishing can sometimes nonrandomly target individuals that are socially connected. For instance, we often preferentially harvest the largest fish, which in some species are also the most socially connected individuals. This type of harvest can radically disrupt the social organization of the remaining fish (Fig. 10.13). We could potentially use social network maps to help design fishing rules to minimize the disruption.
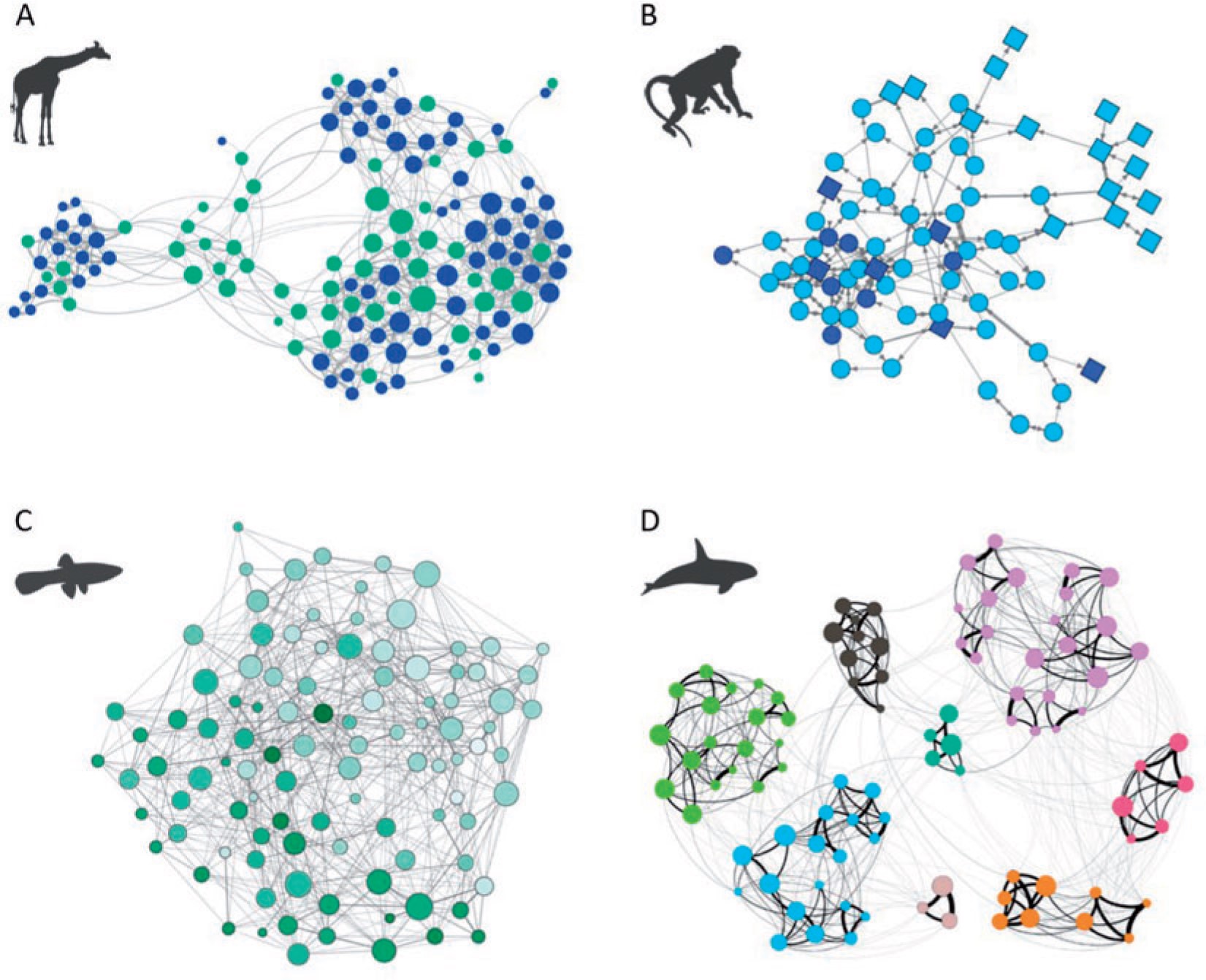
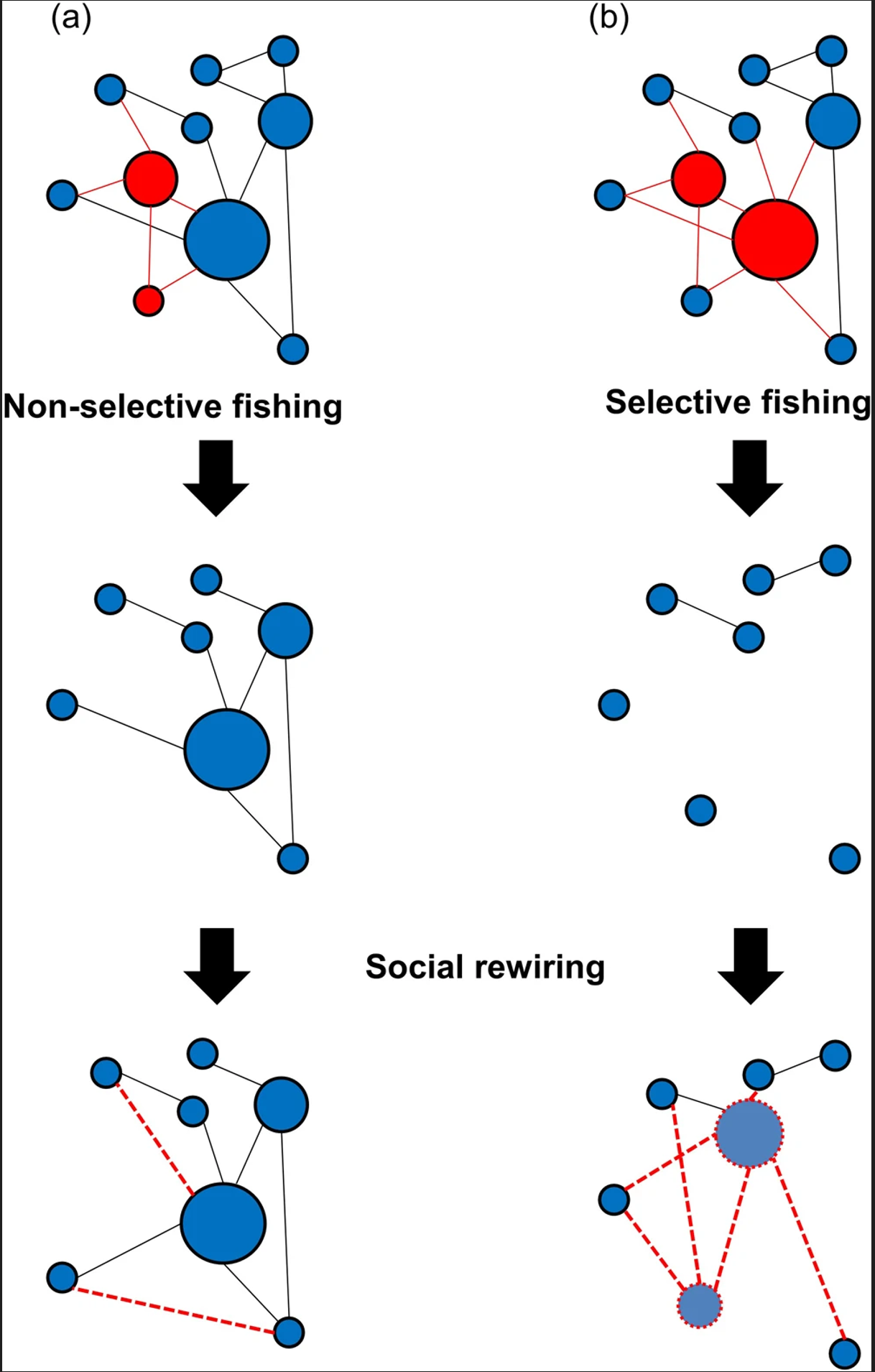
A big roadblock to designing more socially aware conservation practices is our woefully inadequate understanding of how most animal societies are organized. Creating social network maps currently involves years of painstaking direct observations. As a result, we have constructed maps for a relatively small number of species. In 2019, the Animal Social Network Repository had archived social networks for only about 45 species.88 But tools such as automated acoustic monitors and miniaturized cameras fitted to animals will make it easier to collect the data on interpersonal relationships needed to create social network maps.89These systems will also help us monitor how social structure changes. We know that animal societies are dynamic, and their structure can change in response to changing environmental conditions, the loss of socially important individuals, and a range of more inscrutable reasons such as interpersonal grudges that cause social groups to fracture.
Restoring Culture
We are sometimes able to restore aspects of culture in cases where the original culture has been lost or degraded. We have most commonly done this as part of the last-ditch conservation efforts of captive breeding and reintroducing populations. In some of these cases, we can attempt to preserve some of the social structure and cultural knowledge present in wild populations when we establish captive-bred populations or when we translocate individuals to establish a new population. For example, we can use a social network map to identify the social groups present in wild populations and then establish a captive colony or translocate populations as intact social groups instead of as a random collection of individuals. Black-tailed prairie dogs (Cynomys ludovicianus) translocated as part of an intact family group are more likely to survive and reproduce than those released without social connections.90 We are also beginning to do a better job of designing captive breeding programs in ways that maintain social structure and cohesion. This includes being mindful of social interactions when moving individuals between captive colonies and designing enclosures that promote natural social interactions. We can use social network analysis to monitor social conditions during and after reintroduction to ensure that they resemble those in long-standing wild populations. For instance, researchers used social network analysis to show that reintroduced populations of African lions (Panthera leo) that were formed using captive-bred individuals developed socially cohesive interactions that were broadly similar to those observed in an entirely wild pride.91
In most cases, however, it is difficult to preserve social structure as populations go through the ordeal of captive breeding and translocation. For one, social dynamics within a captive-bred enclosure are usually quite different from those in the wild. Captive-bred populations are also isolated from generational traditions as well as the experiential knowledge that wild population gain on a daily basis. As a result, captive-bred individuals may be ill-equipped to negotiate the dynamic challenges of wild life. Those in populations of reintroduced or translocated migratory animals may lack the socially acquired knowledge and skills needed to complete successful migrations—the reintroduced moose and bighorn sheep populations mentioned above are examples. We therefore typically have to try and reconstruct culture to varying degrees when we reintroduce populations. An approach to doing this is to archive animal culture in various ways and use the archive to teach individuals in captive-bred or translocated populations. For instance, captive-bred regent honeyeaters that are tutored using recorded wild regent honeyeater songs have significantly improved survival upon their release than birds that don’t receive tutoring.92 As you might imagine, a recording is often a poor substitute for the real thing. In some cases, we might be able to provide captive-bred or reared individuals with more experienced tutors of their own species. For example, black-tailed prairie dogs (Cynomys ludovicianus) live in social groups that help each other avoid falling victim to a range of predators such as weasels (Mustela spp.) and coyotes (Canis latrans). Like elephants, their predator avoidance skills are complex and dynamically adjust to match the threat provided by each species and the context. They also have a language of calls to communicate the nature of the threat and to organize appropriate responses. A lot (although not all) of this knowledge is socially learned by young prairie dogs as they grow up and is not something that can be easily taught in the absence of that social context. In one study, captive-reared prairie dogs that were trained to avoid predators with the help of an experienced adult female were significantly better at predator avoidance and had higher survival when they were released to the wild than individuals that were trained without the help of an experienced adult.93
When species are critically endangered, there are rarely living individuals in the wild that can act as teachers to captive-bred individuals, and may lack a rich archive of the original wild culture. In these cases, we are forced to take more creative license and provide a surrogate culture of our own design. Probably the best-known examples are our attempts to teach migration skills to captive-reared migratory birds. Whooping cranes (Grus americana) have suffered a severe decline across their North American range. In 1941, there was only one small flock of 15 birds left that migrated between Wood Buffalo National Park in Canada and Aransas National Wildlife Refuge in Texas.94 A captive breeding program was started to help augment numbers in the last wild population, and in 2001, an effort was launched to use the captive-reared individuals to restore a second eastern migratory population. The program began by imprinting young birds to accept humans as elder members of the flock and then leading the birds on training fall migrations from Minnesota to Florida using ultralight aircraft (Fig. 10.14). The birds learned enough along the way to make the return trip to the Minnesota breeding ground on their own.
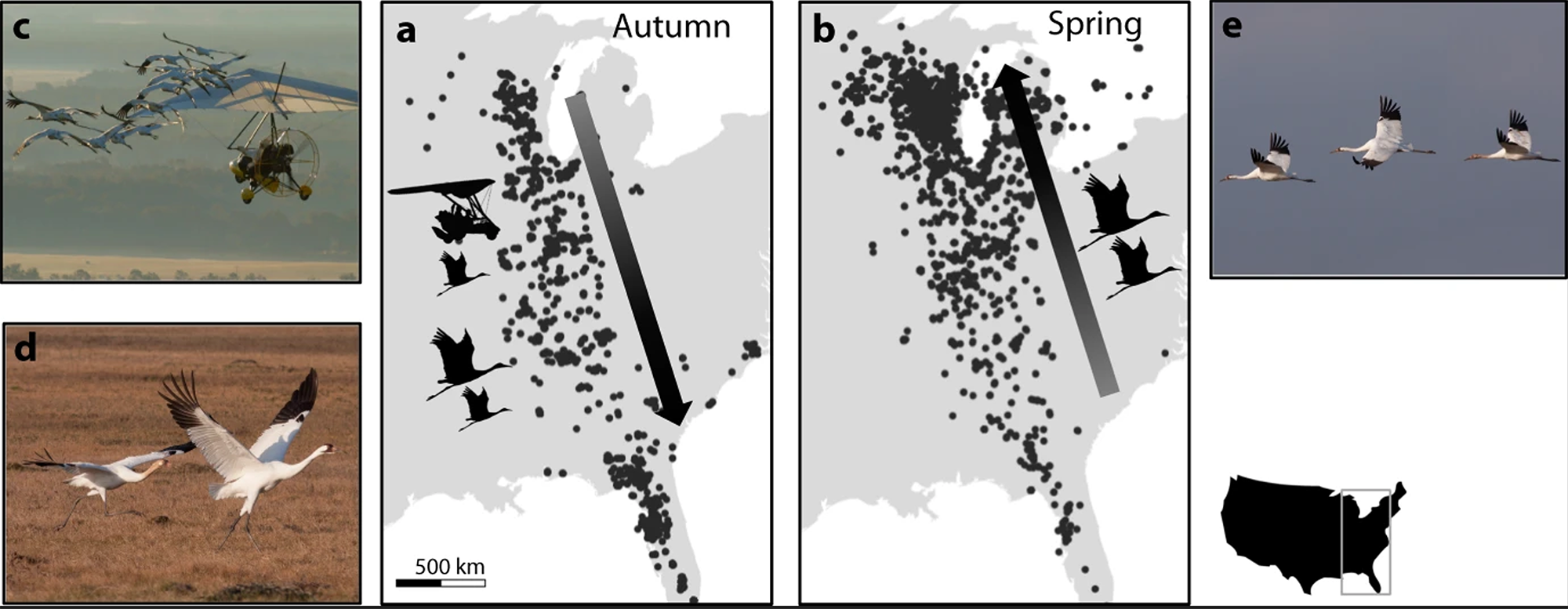
We instinctively knew that we were bad whooping crane teachers—it’s hard to give a master class on something that we are far from a master of. After the number of whooping cranes had increased enough, the hand rearing and ultralight trainings were stopped. Instead, birds were reared by other whooping cranes and released into the wild population to learn the migration from wild birds. Subsequent monitoring has confirmed that we were correct to quickly get out of the business of tutoring birds. Whooping cranes don’t simply follow fixed migration routes. They dynamically adjust the route each year depending on the environmental conditions they encounter. The adjustment involves both social and experiential forms of learning—that is, they get information from other birds as well as from their own assessment of conditions. Interestingly, young birds primarily follow the cue of older birds, while older birds are more likely use their own assessment of the current situation to make adjustments on the fly.95 Those adjustments have included moving the location of the overwintering locations north as climate change creates suitable wintering habitat that is closer to the summer breeding ground.96
The whooping crane example illustrates both the possibilities and limitations of restoring animal culture. We successfully taught whooping cranes a core aspect of being a whooping crane—an essential step in preventing the species from going extinct. But our lesson was rudimentary at best. It also skipped over all the other aspects of whooping crane culture. Much of that rich culture was undoubtedly lost when the population dwindled to those 15 birds. Still, culture is resilient and dynamic. Whooping cranes have rebuilt the cultural knowledge that helps them dynamically adjust their migration route each year.
Modifying Culture
Just as restoring ecosystems to past conditions may not work during the Anthropocene (see Chap. 9), restoring animal culture to what it was in the past may not work, either. Instead, we could modify animal culture in ways that we think will help populations adjust to the range of novel stresses and rapid environmental changes that they will face as the Anthropocene progresses, that is, custom-build Anthropocene cultures. This idea comes with a philosophical can of worms (see Chap. 9), but in some ways, it is not as radical as it sounds. The resilience of whooping crane culture illustrates—just like in our culture—how dynamic and protean animal cultures are. The resilience of culture is one of its most powerful traits during times of change. We might be able to leverage the innate adaptability of culture to nudge it in particularly advantageous directions. For instance, when reintroducing populations of migratory animals, we could train individuals on migration routes that follow climate corridors or that take advantage of wildlife crossings (see Figs. 8.24Figure 8.24. An accessibility retrofit to an incidental wildlife crossing. (A) This culvert runs underneath State Route 118, a two-lane highway in Ventura County, California. It provides safe passage but is largely inaccessible to many animals, particularly smaller ones. Ramps have been installed to improve accessibility. (B) This black bear (Ursus americanus) probably doesn’t need the ramp, but it seems to appreciate it. Source: US National Park Service, https://flic.kr/p/2kSMhSA; b: https://flic.kr/p/2kSKD6Z. and 8.25
Figure 8.25. A wildlife crossing spanning the Trans-Canada Highway in Banff National Park, Canada. This is one of 38 wildlife underpasses and 6 overpasses strategically placed throughout the park. Species including black bears, wolves, coyotes, cougars, moose, elk, and bighorn sheep regularly use the crossings. Source: WikiPedant, https://commons.wikimedia.org/w/index.php?curid=45248553).
We could also use an understanding of social structure to help resolve conflicts between animals and humans or when our actions create conflicts between animals. An example involves California (Zalophus californianus) and Steller (Eumetopias jubatus) sea lions that have discovered that the base of the Bonneville Dam on the Columbia River is a great place to catch salmon. Migrating salmon bunch up at the base of the dam as they wait their turn to go through fish ladders that will get them around the obstacle. Some of the normally coastal sea lions have found the ideal fishing spot and regularly set up shop during salmon migration. The gorging sea lions are an issue because humans have pushed salmon species toward extinction, and the sea lions add another source of mortality. In years when there are a lot of sea lions at the base of the dam, they increase the mortality of Chinook salmon (Oncorhynchus tshawytscha) by 21% compared to years with fewer sea lions.97 To protect the salmon, managers now regularly kill sea lions at the dam to keep their numbers low. But knowledge about the Bonneville Dam fishing hole is socially transmitted through sea lion populations. In the case of California sea lions, social network analysis has revealed that a few key individuals are responsible for communicating information about the dam. Remarkably, that takes place at haul-out sites near the mouth of the river, 335 km away from the dam. If we had understood this social dynamic earlier, we could have targeted culling efforts on the earliest innovators and perhaps prevented knowledge about Bonneville Dam from spreading widely through the sea lion population. Although fishing at Bonneville Dam has now become a widespread aspect of sea lion culture in the region, we might still be able to use the information about the social network to minimize the amount of culling we have to do.98
Creating a Shared Human-Animal Culture
Managers have also tried nonlethal harassment such as loud noises and rubber bullets to drive sea lions away from Bonneville dam. These work briefly, but sea lions soon become wise to the tactics and ignore them.99 This failure says more about us than it does the sea lions. We don’t understand them very well and don’t give them much credit when it comes to their ability to learn, adjust, and outwit us. We have a universal blind spot to relating to the rich lives of organisms other than ourselves. Many conservationists argue that we need to radically adjust our relationship with animals in order to shepherd all of us through the Anthropocene. They argue that we need to recognize animals as equal partners in conservation, not simply as wards of our care.100
There are many examples throughout our shared histories of cooperative interaction with animals to our mutual benefit. In one relatively recent example, bottlenose dolphins (Tursiops truncatus) have formed a long-lasting cooperative fishing relationship with fishermen in southern Brazil. The dolphins chase fish toward fishermen waiting in shallow water with nets. The herding helps the fishermen, and the nets apparently help disorient the fish, making them easier to catch by the dolphins.101 A more cooperative approach might help with biodiversity conservation, but so far we don’t have examples that are as clear-cut as the dolphins and Brazilian fishermen. In some cases, we are attempting to interact with animals on more equal terms. An example is our shifting approach to resolving human-animal conflicts, such as sea lions eating all the fish at the base of a dam or bears breaking into homes looking for food. In the past, we focused on the animal part of the conflict and then only from our own perspective, not theirs. As a result, our efforts were more about pest removal than conflict resolution, particularly in Western and globalized societies. We are now focusing equal attention on our own role in generating and exacerbating conflicts. We are also developing strategies that are focused around fostering healthier relationships with animals.
Our relationship with bears provides an example. In many parts of the world, various species of bear increasingly interact with humans along the wildland-urban interface. The encounters are driven by the expansion of urbanization into bear habitat, the many attractive qualities that urban environments have for bears such as ample food, and environmental changes such as climate change that are degrading the quality of the bear’s more natural habitat. Many of our encounters with bears are positive. You have probably seen videos of bears cavorting in swimming pools (see Additional Resources)—something that is fun for both the bears and us. But our interactions are just as often negative and can result in property damage, unhealthy lifestyles for the bears, and injuries and deaths for the bears, ourselves, and our pets. We have tried managing these conflicts by excluding bears from our living spaces—often by killing them. But in many places, our philosophy has shifted toward trying to cohabitate with bears in positive ways. These management programs focus on educating humans on how to avoid attracting bears into bad situations—such as using bear-proof trash receptacles—and on how to interact with bears so that the encounters don’t degrade into conflict. This involves developing ways of communicating directly with bears in their own terms. A case from the Colorado Front Range provides an example. A homeowner accidently left their garage door open, and curious bears soon found the refrigerator inside. The bears returned the next day and tore off the siding of the now-closed garage door in an effort to get back into the fridge. The situation could have easily devolved into an even worse situation. But the homeowner decided to reason directly with the bears. They moved the refrigerator into the main part of the house and wiped down the garage with ammonia to remove any sent of food and bears. They also left the garage door open for a few days to show the bears that the food source was gone. The bears came by one more time to investigate, but then never entered the garage again.102
Managers also now interact with and evaluate bears as individuals that have distinct histories, personal situations, and personalities. Those individual circumstances help inform the best form of intervention in cases where conflicts arise. Many negative human-bear interactions stem from idiosyncratic breakdowns in communication, such as humans inadvertently attracting bears with food. In most cases, these conflicts can be resolved by better communication, as evidenced in the Colorado case. Also, some interactions are driven by unusual or temporary circumstances such as a drought or wildfire that reduces the availability of food in the bear’s natural habitat. In these situations, managers have provided supplemental food to keep bears from wandering into urbanized areas in search of it.103 It is only in rare circumstances that a bear has a personality or life circumstance that causes repeated or dangerous conflicts with humans. We are getting better at identifying the truly troubled cases. In one instance, managers used forensic DNA analysis to exonerate a black bear (Ursus americanus)—named Hank by the human residents—that had been accused of breaking into a series of houses in the Lake Tahoe area. Officials had been searching for Hank and likely would have killed him because of his repeated extremely dangerous behavior. But the DNA evidence proved that Hank was innocent; the break-ins were caused by several other bears.104
Humans used to have this type of nuanced and personalized relationship with other species. Many Indigenous cultures still do. But as our numbers grew and our ability to use and manipulate the natural world expanded, the more distant our relationships with other species became. Most of us today have a largely impersonal, narrow, and often superficial interaction with nature. Precisely because we now have the power to radically change life on Earth and even to wipe out large chunks of it without too much thought, we need to rebuild our relationship with the natural world. We need to reconcile our life on Earth.
Chapter Summary
Super Ecosystems Last All Summer Long
The fields of conservation biology and ecological restoration provide tools and techniques for reducing our impacts on the Earth System, mitigating their negative effects, and repairing the damage. But as the pace and magnitude of Anthropocene change continue to increase, we may need to design and promote the development of Anthropocene-adapted ecosystems.
- Rapidly emerging biotechnology is improving our ability to describe patterns of genomic variation, to understand how genomes and the environment interact to produce functional traits, and to directly manipulate the genomes and functional traits of organisms.
- Applications of these techniques to biodiversity conservation include predicting how wild populations will respond to climate change (Figure 10.2), helping to adapt populations to climate change, managing invasive populations (Figure 10.3), and preserving the germplasm of endangered populations (Figures 10.4, 10.5).
- We can move organism to aid biodiversity conservation by reintroducing native populations to regions where they have gone locally extinct and also potentially by introducing non-native populations that are ecological analogs to extinct native species (Figure 10.6). We might be able to help organisms move in response to climate change by creating climate corridors and refugia or by actively moving populations to locations with more suitable climate.
- We might be able to help populations evolutionarily adapt to changing Anthropocene conditions. Current and potential approaches include captive breeding and genetic rescue (Figure 10.7), augmenting the population genetic diversity available to natural selection (Figure 10.8), and selective breeding and genome editing (Figure 10.9).
- We might be able to acclimatize populations to changing conditions by using epigenetic mechanisms or by manipulating the microbiome of organisms.
- We have underappreciated the social aspects of biodiversity (Table 10.1). We need to take more active steps to understand and preserve animal cultures and the social connections within populations (Figures 10.11, 10.12).
- A better understanding of animal cultures and social relationships will help us reduce disruptions caused by our activities (Figure 10.13), repair or restore cultures (Figure 10.14), and even modify both animal and human cultures to create more mutually beneficial relationships.
Additional Resources
- This short film by the Wildlife Conservation Society tells the history of the bathysphere and the Nonsuch expedition: YouTube.
- Plumer, B., Barclay, E., Belluz, J., Irfan, U., 2018, A simple guide to CRISPR, one of the biggest science stories of the decade. Vox, December 27, 2018, Vox Media: A Simple Guide to CRISPR. This article provides an overview of CRISPR.
- How to Clone a Mammoth: The Science of De-Extinction, by Beth Shapiro (Princeton, NJ: Princeton University Press, 2020), explores the possibility of resurrecting extinct species.
- Even if you are not a bird aficionado, you can probably hear the song differences among these three white-crowned sparrow populations from Marin County, California.
- Bolinas: Bolinas Sparrow Song, SoundCloud
- Abbott’s Lagoon: Abbott’s Lagoon Sparrow, SoundCloud
- Schooner Bay: Schooner Bay Sparrow, SoundCloud
- Also, the following article by Kim Todd explores how the songs of white-crowned sparrows in the San Francisco Bay Region have been changing: Sparrows lose their language with urban noise. Bay Nature Magazine (January-March 2016): https://baynature.org/article/the-language-of-sparrows/
- Check out these videos of dolphins using shells (YouTube) and sponges (YouTube) as tools to capture prey.
- A short video of regent honeyeaters who have learned another species’ song: YouTube.
- A family of black bears have a pool party in suburban New Jersey: YouTube.
References
*Abrahms, B., Teitelbaum, C.S., Mueller, T., Converse, S.J., 2021. Ontogenetic shifts from social to experiential learning drive avian migration timing. Nat. Commun. 12, 7326. https://doi.org/10.1038/s41467-021-27626-5
79Aikens, E.O., Kauffman, M.J., Merkle, J.A., Dwinnell, S.P.H., Fralick, G.L., Monteith, K.L., 2017. The greenscape shapes surfing of resource waves in a large migratory herbivore. Ecol. Lett. 20, 741–50. https://doi.org/10.1111/ele.12772
* Allen, C.R.B., Brent, L.J.N., Motsentwa, T., Weiss, M.N., Croft, D.P., 2020. Importance of old bulls: leaders and followers in collective movements of all-male groups in African savannah elephants (Loxodonta africana). Sci. Rep. 10, 13996. https://doi.org/10.1038/s41598-020-70682-y
11Auster, P.J., Hodge, B.C., McKee, M.P., Kraus, S.D., 2020. A scientific basis for designation of the Northeast Canyons and Seamounts Marine National Monument. Front. Mar. Sci. 7. https://doi.org/10.3389/fmars.2020.00566
54Baums, I.B., Baker, A.C., Davies, S.W., Grottoli, A.G., Kenkel, C.D., Kitchen, S.A., Kuffner, I.B., LaJeunesse, T.C., Matz, M.V., Miller, M.W., Parkinson, J.E., Shantz, A.A., 2019. Considerations for maximizing the adaptive potential of restored coral populations in the western Atlantic. Ecol. Appl. 29, e01978. https://doi.org/10.1002/eap.1978
13Beebe, W., 1934. Half Mile Down. San Diego, CA: Harcourt Brace.
73Bilas, R.D., Bretman, A., Bennett, T., 2021. Friends, neighbours and enemies: an overview of the communal and social biology of plants. Plant Cell Environ. 44, 997–1013. https://doi.org/10.1111/pce.13965
12Billett, D.S.M., Jones, D.O.B., Weaver, P.P.E., 2019. Improving environmental management practices in deep-sea mining. In R. Sharma (Ed.), Environmental Issues of Deep-Sea Mining: Impacts, Consequences and Policy Perspectives. Cham, Switzerland: Springer International, 403–46. https://doi.org/10.1007/978-3-030-12696-4_15
102Boonman-Berson, S., Turnhout, E., Carolan, M., 2016. Common sensing: human-black bear cohabitation practices in Colorado. Geoforum 74, 192–201. https://doi.org/10.1016/j.geoforum.2016.06.010
31Borges, A.A., Pereira, A.F., 2019. Potential role of intraspecific and interspecific cloning in the conservation of wild mammals. Zygote 27, 111–17. https://doi.org/10.1017/S0967199419000170
*Brask, J.B., Ellis, S., Croft, D.P., 2021. Animal social networks: an introduction for complex systems scientists. J. Complex Networks 9, cnab001. https://doi.org/10.1093/comnet/cnab001
18Breed, M.F., Harrison, P.A., Blyth, C., Byrne, M., Gaget, V., Gellie, N.J.C., Groom, S.V.C., Hodgson, R., Mills, J.G., Prowse, T.A.A., Steane, D.A., Mohr, J.J., 2019. The potential of genomics for restoring ecosystems and biodiversity. Nat. Rev. Genet. 20, 615–28. https://doi.org/10.1038/s41576-019-0152-0
104California Department of Fish and Wildlife, 2022. DNA evidence prompts revised response to Tahoe Keys bear incidents. February 24, 2022, https://wildlife.ca.gov/Conservation/Mammals/Black-Bear/Blog/dna-evidence-prompts-revised-response-to-tahoe-keys-bear-incidents.
71Chakravarti, L.J., van Oppen, M.J.H., 2018. Experimental evolution in coral photosymbionts as a tool to increase thermal tolerance. Front. Mar. Sci. 5. https://doi.org/10.3389/fmars.2018.00227
60Clark, S.L., Schlarbaum, S.E., Saxton, A.M., Baird, R., 2019. Eight-year blight (Cryphonectria parasitica) resistance of backcross-generation American chestnuts (Castanea dentata) planted in the southeastern United States. For. Ecol. Manage. 433, 153–61. https://doi.org/10.1016/j.foreco.2018.10.060
66Cleves, P.A., Strader, M.E., Bay, L.K., Pringle, J.R., Matz, M.V., 2018. CRISPR/Cas9-mediated genome editing in a reef-building coral. Proc. Natl. Acad. Sci. 115, 5235–40. https://doi.org/10.1073/pnas.1722151115
82Crates, R., Langmore, N., Ranjard, L., Stojanovic, D., Rayner, L., Ingwersen, D., Heinsohn, R., 2021. Loss of vocal culture and fitness costs in a critically endangered songbird. Proc. R. Soc. B Biol. Sci. 288, 20210225. https://doi.org/10.1098/rspb.2021.0225
75* Danchin, E., Nöbel, S., Pocheville, A., Dagaeff, A.-C., Demay, L., Alphand, M., Ranty-Roby, S., van Renssen, L., Monier, M., Gazagne, E., Allain, M., Isabel, G., 2018. Cultural flies: conformist social learning in fruitflies predicts long-lasting mate-choice traditions. Science 362, 1025–30. https://doi.org/10.1126/science.aat1590
13Da Ros, Z., Dell’Anno, A., Morato, T., Sweetman, A.K., Carreiro-Silva, M., Smith, C.J., Papadopoulou, N., Corinaldesi, C., Bianchelli, S., Gambi, C., Cimino, R., Snelgrove, P., Van Dover, C.L., Danovaro, R., 2019. The deep sea: the new frontier for ecological restoration. Mar. Policy 108, 103642. https://doi.org/10.1016/j.marpol.2019.103642
36D’Elia, J., Haig, S.M., Mullins, T.D., Miller, M.P., 2016. Ancient DNA reveals substantial genetic diversity in the California Condor (Gymnogyps californianus) prior to a population bottleneck. The Condor 118, 703–14. https://doi.org/10.1650/CONDOR-16-35.1
38Donlan, C., Berger, J., Bock, C.E., Bock, J.H., Burney, D.A., Estes, J.A., Foreman, D., Martin, P.S., Roemer, G.W., Smith, F.A., Soulé, M.E., Greene, H.W., 2006. Pleistocene rewilding: an optimistic agenda for twenty‐first century conservation. Am. Nat. 168, 660–81. https://doi.org/10.1086/508027
91Dunston, E.J., Abell, J., Doyle, R.E., Kirk, J., Hilley, V.B., Forsyth, A., Jenkins, E., Freire, R., 2017. An assessment of African lion Panthera leo sociality via social network analysis: prerelease monitoring for an ex situ reintroduction program. Curr. Zool. 63, 301–11. https://doi.org/10.1093/cz/zow012
5Ebert, D.A., Papastamatiou, Y.P., Kajiura, S.M., Wetherbee, B.M., 2017. Etmopterus lailae sp. nov., a new lanternshark (Squaliformes: Etmopteridae) from the northwestern Hawaiian Islands. Zootaxa 4237, zootaxa.4237.2.10. https://doi.org/10.11646/zootaxa.4237.2.10
100Edelblutte, É., Krithivasan, R., Hayek, M.N., 2022. Animal agency in wildlife conservation and management. Conserv. Biol., e13853. https://doi.org/10.1111/cobi.13853
*Edesi, J., Tolonen, J., Ruotsalainen, A.L., Aspi, J., Häggman, H., 2020. Cryopreservation enables long-term conservation of critically endangered species Rubus humulifolius. Biodiversity Conserv. 29, 303–14. https://doi.org/10.1007/s10531-019-01883-9
9Elton, C.S. 1958. The Ecology of Invasions by Plants and Animals. North Yorkshire, UK: Methuen.
27Esvelt, K.M., Gemmell, N.J., 2017. Conservation demands safe gene drive. PLoS Biol. 15, e2003850. https://doi.org/10.1371/journal.pbio.2003850
* Fiorito, G., Scotto, P., 1992. Observational learning in Octopus vulgaris. Science 256, 545–47. https://doi.org/10.1126/science.256.5056.545
49Galla, S.J., Moraga, R., Brown, L., Cleland, S., Hoeppner, M.P., Maloney, R.F., Richardson, A., Slater, L., Santure, A.W., Steeves, T.E., 2020. A comparison of pedigree, genetic and genomic estimates of relatedness for informing pairing decisions in two critically endangered birds: implications for conservation breeding programmes worldwide. Evol. Appl. 13, 991–1008. https://doi.org/10.1111/eva.12916
78Garland, E.C., McGregor, P.K., 2020. Cultural transmission, evolution, and revolution in vocal displays: insights from bird and whale song. Front. Psychol. 11. https://doi.org/10.3389/fpsyg.2020.544929
* Garland, E.C., Goldizen, A.W., Rekdahl, M.L., Constantine, R., Garrigue, C., Hauser, N.D., Poole, M.M., Robbins, J., Noad, M.J., 2011. Dynamic horizontal cultural transmission of humpback whale song at the ocean basin scale. Curr. Biol. 21, 687–91. https://doi.org/10.1016/j.cub.2011.03.019
103Garshelis, D.L., Baruch-Mordo, S., Bryant, A., Gunther, K.A., Jerina, K., 2017. Is diversionary feeding an effective tool for reducing human–bear conflicts? Case studies from North America and Europe. Ursus 28, 31–55. https://doi.org/10.2192/URSU-D-16-00019.1
10Glover, A.G., Smith, C.R., 2003. The deep-sea floor ecosystem: current status and prospects of anthropogenic change by the year 2025. Environ. Conserv. 30, 219–41. https://doi.org/10.1017/S0376892903000225
48Gómez-Mendoza, L., Arriaga, L., 2007. Modeling the effect of climate change on the distribution of oak and pine species of Mexico. Conserv. Biol. 21, 1545–55.
47Gómez-Ruiz, P.A., Sáenz-Romero, C., Lindig-Cisneros, R., 2020. Early performance of two tropical dry forest species after assisted migration to pine–oak forests at different altitudes: strategic response to climate change. J. Forest. Res. 31, 1215–23. https://doi.org/10.1007/s11676-019-00973-2
4Govenar, B., 2012. Energy transfer through food webs at hydrothermal vents: linking the lithosphere to the biosphere. Oceanography 25, 246–55.
39Griffiths, C.J., Jones, C.G., Hansen, D.M., Puttoo, M., Tatayah, R.V., Müller, C.B., Harris, S., 2010. The use of extant non-Indigenous tortoises as a restoration tool to replace extinct ecosystem engineers. Restor. Ecol. 18, 1–7. https://doi.org/10.1111/j.1526-100X.2009.00612.x
25Grognet, P., Lalucque, H., Malagnac, F., Silar, P., 2014. Genes that bias Mendelian segregation. PLoS Genet. 10, e1004387. https://doi.org/10.1371/journal.pgen.1004387
40Hansen, D.M., Donlan, C.J., Griffiths, C.J., Campbell, K.J., 2010. Ecological history and latent conservation potential: large and giant tortoises as a model for taxon substitutions. Ecography 33, 272–84. https://doi.org/10.1111/j.1600-0587.2010.06305.x
70Howells, E.J., Beltran, V.H., Larsen, N.W., Bay, L.K., Willis, B.L., van Oppen, M.J.H., 2012. Coral thermal tolerance shaped by local adaptation of photosymbionts. Nat. Clim. Change 2, 116–20. https://doi.org/10.1038/nclimate1330
14Jamieson, A.J., Malkocs, T., Piertney, S.B., Fujii, T., Zhang, Z., 2017. Bioaccumulation of persistent organic pollutants in the deepest ocean fauna. Nat. Ecol. Evol. 1, 0051. https://doi.org/10.1038/s41559-016-0051
80Jesmer, B.R., Merkle, J.A., Goheen, J.R., Aikens, E.O., Beck, J.L., Courtemanch, A.B., Hurley, M.A., McWhirter, D.E., Miyasaki, H.M., Monteith, K.L., Kauffman, M.J., 2018. Is ungulate migration culturally transmitted? Evidence of social learning from translocated animals. Science 361, 1023–25. https://doi.org/10.1126/science.aat0985
50Johnson, W.E., Onorato, D.P., Roelke, M.E., Land, E.D., Cunningham, M., Belden, R.C., McBride, R., Jansen, D., Lotz, M., Shindle, D., Howard, J., Wildt, D.E., Penfold, L.M., Hostetler, J.A., Oli, M.K., O’Brien, S.J., 2010. Genetic restoration of the Florida panther. Science 329, 1641–45. https://doi.org/10.1126/science.1192891
21Julian, C.G., 2017. Epigenomics and human adaptation to high altitude. J. Appl. Physiol. 123, 1362–70. https://doi.org/10.1152/japplphysiol.00351.2017
52Kasperbauer, T.J., 2017. Should we bring back the passenger pigeon? The ethics of de-extinction. Ethics Policy Environ. 20, 1–14. https://doi.org/10.1080/21550085.2017.1291831
* Kelly, T.R., Kimball, M.G., Stansberry, K.R., Lattin, C.R., 2020. No, you go first: phenotype and social context affect house sparrow neophobia. Biol. Lett. 16, 20200286. https://doi.org/10.1098/rsbl.2020.0286
85Kühl, H.S., Boesch, C., Kulik, L., Haas, F., Arandjelovic, M., Dieguez, P., Bocksberger, G., McElreath, M.B., Agbor, A., Angedakin, S., Ayimisin, E.A., Bailey, E., Barubiyo, D., Bessone, M., Brazzola, G., Chancellor, R., Cohen, H., Coupland, C., Danquah, E., Deschner, T., Dowd, D., Dunn, A, Egbe, E.E., Eshuis, H., Goedmakers, A, Granjon, A.1, Head, J., Hedwig, D., Hermans,V., Imong, I., Jeffery, K., Jones, S., Junker, J., Kadam, P., Kambere, M., Kambi, M., Kienast, I., Kujirakwinja, D., Langergraber, K., Lapuente, J., Larson, B., Lee, K. Kevin, Leinert, V., Llana, M., Maretti, G, Marrocol, S, Martin, R., Mbi, J.T., Meier, A.C., Morgan, B., Morgan, D., Mulindahabi, F., Murai, M., Neil, E, Niyigaba, P, Ormsby, L.J., Orume, R., Pacheco, L., Piel, A., Preece, J., Regnaut, S., Rundus, A, Sanz, C., van Schijndell, J., Sommer, Volker, V., Stewart, F., Tagg, N., Vendras, E, Vergnes, V.3, Welsh, A., Wessling, E.G., Willie, J., Wittig, R.M.Roman, Yuh, Y.G., Yurkiw, K., Zuberbühler, K., Kalan, A.K. 2019. Human impact erodes chimpanzee behavioral diversity. Science 363, 1453–55. https://doi.org/10.1126/science.aau4532
26Leitschuh, C.M., Kanavy, D., Backus, G.A., Valdez, R.X., Serr, M., Pitts, E.A., Threadgill, D., Godwin, J., 2018. Developing gene drive technologies to eradicate invasive rodents from islands. J. Responsible Innovation 5, S121–S138. https://doi.org/10.1080/23299460.2017.1365232
33Marr, M.M., Brace, S., Schreve, D.C., Barnes, I., 2018. Identifying source populations for the reintroduction of the Eurasian beaver, Castor fiber L. 1758, into Britain: evidence from ancient DNA. Sci. Rep. 8, 2708. https://doi.org/10.1038/s41598-018-21173-8
32Marshall, C., 2020. Beaver families win legal “right to remain.” BBC News, August 6, 2020. https://www.bbc.com/news/science-environment-53658375.
Monterey Bay Aquarium Research Institute. (2015). Autonomous underwater vehicles. Accessed November 30, 2015, https://www.mbari.org/at-sea/vehicles/autonomous-underwater-vehicles/.
43McGuire, J.L., Lawler, J.J., McRae, B.H., Nuñez, T.A., Theobald, D.M., 2016. Achieving climate connectivity in a fragmented landscape. Proc. Natl. Acad. Sci. 113, 7195–200. https://doi.org/10.1073/pnas.1602817113
55Miller, M.W., Baums, I.B., Pausch, R.E., Bright, A.J., Cameron, C.M., Williams, D.E., Moffitt, Z.J., Woodley, C.M., 2018. Clonal structure and variable fertilization success in Florida Keys broadcast-spawning corals. Coral Reefs 37, 239–49. https://doi.org/10.1007/s00338-017-1651-0
41Morelli, T.L., Barrows, C.W., Ramirez, A.R., Cartwright, J.M., Ackerly, D.D., Eaves, T.D., Ebersole, J.L., Krawchuk, M.A., Letcher, B.H., Mahalovich, M.F., Meigs, G.W., Michalak, J.L., Millar, C.I., Quiñones, R.M., Stralberg, D., Thorne, J.H., 2020. Climate-change refugia: biodiversity in the slow lane. Front. Ecol. Environ. 18, 228–34. https://doi.org/10.1002/fee.2189
87Morrison, R.E., Groenenberg, M., Breuer, T., Manguette, M.L., Walsh, P.D., 2019. Hierarchical social modularity in gorillas. Proc. R. Soc. B Biol. Sci. 286, 20190681. https://doi.org/10.1098/rspb.2019.0681
67Muñiz-Castillo, A.I., Rivera-Sosa, A., Chollett, I., Eakin, C.M., Andrade-Gómez, L., McField, M., Arias-González, J.E., 2019. Three decades of heat stress exposure in Caribbean coral reefs: a new regional delineation to enhance conservation. Sci. Rep. 9, 11013. https://doi.org/10.1038/s41598-019-47307-0
37Norris, K., Terry, A., Hansford, J.P., Turvey, S.T., 2020. Biodiversity conservation and the Earth System: mind the gap. Trends Ecol. Evol. 35, 919–26. https://doi.org/10.1016/j.tree.2020.06.010
53Norström, A.V., Nyström, M., Jouffray, J.-B., Folke, C., Graham, N.A., Moberg, F., Olsson, P., Williams, G.J., 2016. Guiding coral reef futures in the Anthropocene. Front. Ecol. Environ. 14, 490–98. https://doi.org/10.1002/fee.1427
8Odum, H.T., Odum, E.P., 1955. Trophic structure and productivity of a windward coral reef community on Eniwetok Atoll. Ecol. Monogr. 25, 291–320. https://doi.org/10.2307/1943285
84Papale, E.B., Azzolin, M.A., Cascão, I., Gannier, A., Lammers, M.O., Martin, V.M., Oswald, J.N., Perez-Gil, M., Prieto, R., Silva, M.A., Torri, M., Giacoma, C., 2021. Dolphin whistles can be useful tools in identifying units of conservation. BMC Zool. 6, 22. https://doi.org/10.1186/s40850-021-00085-7
44Parks, S.A., Carroll, C., Dobrowski, S.Z., Allred, B.W., 2020. Human land uses reduce climate connectivity across North America. Global Change Biol. 26, 2944–55. https://doi.org/10.1111/gcb.15009
46Pedrono, M., Griffiths, O.L., Clausen, A., Smith, L.L., Griffiths, C.J., Wilmé, L., Burney, D.A., 2013. Using a surviving lineage of Madagascar’s vanished megafauna for ecological restoration. Biol. Conserv. 159, 501–6. https://doi.org/10.1016/j.biocon.2012.11.027
*Powell, W., 2016. New genetically engineered American chestnut will help restore the decimated, iconic tree. The Conversation, January 19, 2016. http://theconversation.com/new-genetically-engineered-american-chestnut-will-help-restore-the-decimated-iconic-tree-52191.
*Powell, W.A., Newhouse, A.E., Coffey, V., 2019. Developing blight-tolerant American chestnut trees. Cold Spring Harbor Perspect. Biol. 11, a034587. https://doi.org/10.1101/cshperspect.a034587
63Quigley, K.M., Bay, L.K., van Oppen, M.J.H., 2020a. Genome-wide SNP analysis reveals an increase in adaptive genetic variation through selective breeding of coral. Mol. Ecol. 29, 2176–88. https://doi.org/10.1111/mec.15482
Quigley, K.M., Randall, C.J., van Oppen, M.J.H., Bay, L.K., 2020b. Assessing the role of historical temperature regime and algal symbionts on the heat tolerance of coral juveniles. Biol. Open 9. https://doi.org/10.1242/bio.047316
56Randall, C.J., Negri, A.P., Quigley, K.M., Foster, T., Ricardo, G.F., Webster, N.S., Bay, L.K., Harrison, P.L., Babcock, R.C., Heyward, A.J., 2020. Sexual production of corals for reef restoration in the Anthropocene. Mar. Ecol. Prog. Ser. 635, 203–32. https://doi.org/10.3354/meps13206
15Regalado, A., 2020. China’s BGI says it can sequence a genome for just $100. MIT Technology Review, February 26, 2020. https://www.technologyreview.com/2020/02/26/905658/china-bgi-100-dollar-genome/.
Revive and Restore, 2022. The great passenger pigeon comeback. Accessed September 18, 2022, https://reviverestore.org/about-the-passenger-pigeon/.
23Rey, O., Eizaguirre, C., Angers, B., Baltazar‐Soares, M., Sagonas, K., Prunier, J.G., Blanchet, S., 2020. Linking epigenetics and biological conservation: towards a conservation epigenetics perspective. Funct. Ecol. 34, 414–27. https://doi.org/10.1111/1365-2435.13429
57Rinkevich, B., 2019. Coral chimerism as an evolutionary rescue mechanism to mitigate global climate change impacts. Global Change Biol. 25, 1198–206. https://doi.org/10.1111/gcb.14576
35Robinson, J.A., Bowie, R.C.K., Dudchenko, O., Aiden, E.L., Hendrickson, S.L., Steiner, C.C., Ryder, O.A., Mindell, D.P., Wall, J.D., 2021. Genome-wide diversity in the California condor tracks its prehistoric abundance and decline. Curr. Biol. 31, 2939-2946.e5. https://doi.org/10.1016/j.cub.2021.04.035
88Sah, P., Méndez, J.D., Bansal, S., 2019. A multi-species repository of social networks. Sci. Data 6, 44. https://doi.org/10.1038/s41597-019-0056-z
24Samuel, M.D., Liao, W., Atkinson, C.T., LaPointe, D.A., 2020. Facilitated adaptation for conservation—can gene editing save Hawaii’s endangered birds from climate driven avian malaria? Biol. Conserv. 241, 108390. https://doi.org/10.1016/j.biocon.2019.108390
34San Diego Zoo, 2021. California condor (Gymnogyps californianus) fact sheet: population and conservation status. Last updated November 2, 2021, https://ielc.libguides.com/sdzg/factsheets/californiacondor/population.
98Schakner, Z.A., Buhnerkempe, M.G., Tennis, M.J., Stansell, R.J., van der Leeuw, B.K., Lloyd-Smith, J.O., Blumstein, D.T., 2016. Epidemiological models to control the spread of information in marine mammals. Proc. R. Soc. B Biol. Sci. 283, 20162037. https://doi.org/10.1098/rspb.2016.2037
58Schoepf, V., Carrion, S.A., Pfeifer, S.M., Naugle, M., Dugal, L., Bruyn, J., McCulloch, M.T., 2019. Stress-resistant corals may not acclimatize to ocean warming but maintain heat tolerance under cooler temperatures. Nat. Commun. 10, 4031. https://doi.org/10.1038/s41467-019-12065-0
59Selmoni, O., Lecellier, G., Magalon, H., Vigliola, L., Benzoni, F., Peignon, C., Joost, S., Berteaux-Lecellier, V., 2020. Seascape genomics reveals candidate molecular targets of heat stress adaptation in three coral species. BioRxiv, 2020.05.12.090050. https://doi.org/10.1101/2020.05.12.090050
*Shannon, G., Cordes, L.S., Slotow, R., Moss, C., McComb, K., 2022. Social disruption impairs predatory threat assessment in African elephants. Animals 12, 495. https://doi.org/10.3390/ani12040495
93Shier, D.M., 2006. Effect of family support on the success of translocated black-tailed prairie dogs. Conserv. Biol. 20, 1780–90. https://doi.org/10.1111/j.1523-1739.2006.00512.x
Shier, D.M., Owings, D.H., 2007. Effects of social learning on predator training and postrelease survival in juvenile black-tailed prairie dogs, Cynomys ludovicianus. Anim. Behav. 73, 567–77. https://doi.org/10.1016/j.anbehav.2006.09.009
101Simões-Lopes, P.C., Daura-Jorge, F.G., Cantor, M., 2016. Clues of cultural transmission in cooperative foraging between artisanal fishermen and bottlenose dolphins, Tursiops truncatus (Cetacea: Delphinidae). Zoologia (Curitiba) 33. https://doi.org/10.1590/S1984-4689zool-20160107
97Sorel, M.H., Zabel, R.W., Johnson, D.S., Wargo Rub, A.M., Converse, S.J., 2021. Estimating population-specific predation effects on Chinook salmon via data integration. J. Appl. Ecol. 58, 372–81. https://doi.org/10.1111/1365-2664.13772
45Stralberg, D., Carroll, C., Nielsen, S.E., 2020. Toward a climate-informed North American protected areas network: incorporating climate-change refugia and corridors in conservation planning. Conserv. Lett. 13, e12712. https://doi.org/10.1111/conl.12712
28Sudweeks, J., Hollingsworth, B., Blondel, D.V., Campbell, K.J., Dhole, S., Eisemann, J.D., Edwards, O., Godwin, J., Howald, G.R., Oh, K.P., Piaggio, A.J., Prowse, T.A.A., Ross, J. V., Saah, J.R., Shiels, A.B., Thomas, P.Q., Threadgill, D.W., Vella, M.R., Gould, F., Lloyd, A.L., 2019. Locally fixed alleles: a method to localize gene drive to island populations. Sci. Rep. 9, 15821. https://doi.org/10.1038/s41598-019-51994-0
*Supple, M.A., Shapiro, B., 2018. Conservation of biodiversity in the genomics era. Genome Biol. 19, 131. https://doi.org/10.1186/s13059-018-1520-3
*Supple, M.A., Bragg, J.G., Broadhurst, L.M., Nicotra, A.B., Byrne, M., Andrew, R.L., Widdup, A., Aitken, N.C., Borevitz, J.O., 2018. Landscape genomic prediction for restoration of a Eucalyptus foundation species under climate change. ELife 7, e31835. https://doi.org/10.7554/eLife.31835
96Teitelbaum, C.S., Converse, S.J., Fagan, W.F., Böhning-Gaese, K., O’Hara, R.B., Lacy, A.E., Mueller, T., 2016. Experience drives innovation of new migration patterns of whooping cranes in response to global change. Nat. Commun. 7, 12793. https://doi.org/10.1038/ncomms12793
22Thorson, J.L.M., Smithson, M., Beck, D., Sadler-Riggleman, I., Nilsson, E., Dybdahl, M., Skinner, M.K., 2017. Epigenetics and adaptive phenotypic variation between habitats in an asexual snail. Sci. Rep. 7, 1–11. https://doi.org/10.1038/s41598-017-14673-6
99Tidwell, K.S., Carrothers, B.A., Blumstein, D.T., Schakner, Z.A., 2021. Steller sea lion (Eumetopias jubatus) response to non-lethal hazing at Bonneville Dam. Front. Conserv, Sci. 2. https://www.frontiersin.org/articles/10.3389/fcosc.2021.760866
20Tobi, E.W., Slieker, R.C., Luijk, R., Dekkers, K.F., Stein, A.D., Xu, K.M., Consortium, B.I.O. S., Slagboom, P.E., Zwet, E.W., van Lumey, L.H., Heijmans, B.T., 2018. DNA methylation as a mediator of the association between prenatal adversity and risk factors for metabolic disease in adulthood. Sci. Adv. 4, eaao4364. https://doi.org/10.1126/sciadv.aao4364
92Tripovich, J.S., Popovic, G., Elphinstone, A., Ingwersen, D., Johnson, G., Schmelitschek, E., Wilkin, D., Taylor, G., Pitcher, B.J., 2021. Born to be wild: evaluating the zoo-based regent honeyeater breed for release program to optimise individual success and conservation outcomes in the wild. Front. Conserv. Sci. 2. https://www.frontiersin.org/article/10.3389/fcosc.2021.669563
2Tunesi, L., 2017. Remembering the Bathysphere, a steel ball that changed the course of ocean exploration. Atlas Obscura, August 16, 2017. http://www.atlasobscura.com/articles/bathysphere.
86United Nations Environment Programme, 2018. Report of the CMS Workshop on Conservation Implications of Animal Culture and Social Complexity. Presented at the Third Meeting of the Sessional Committee of the CMS Scientific Council (ScC-SC3), Bonn, May 29 to June 1, 2018. https://www.cms.int/en/document/report-cms-workshop-conservation-implications-animal-culture-and-social-complexity.
94US Fish and Wildlife Service, 2022. Whooping crane. Retrieved July 11, 2022, https://www.fws.gov/species/whooping-crane-grus-americana.
72van Oppen, M.J.H., Blackall, L.L., 2019. Coral microbiome dynamics, functions and design in a changing world. Nat. Rev. Microbiol. 17, 557–67. https://doi.org/10.1038/s41579-019-0223-4
van Oppen, M.J.H., Oliver, J.K., Putnam, H.M., Gates, R.D., 2015. Building coral reef resilience through assisted evolution. Proc. Natl. Acad. Sci. 112, 2307–13. https://doi.org/10.1073/pnas.1422301112
89*Villegas-Ríos, D., Jacoby, D.M.P., Mourier, J., 2022. Social networks and the conservation of fish. Commun. Biol. 5, 1–8. https://doi.org/10.1038/s42003-022-03138-w
17Watsa, M., Erkenswick, G.A., Pomerantz, A., Prost, S., 2020. Portable sequencing as a teaching tool in conservation and biodiversity research. PLoS Biol. 18, e3000667. https://doi.org/10.1371/journal.pbio.3000667
7Watson, J.D., Crick, F.H.C., 1953. Molecular structure of nucleic acids: a structure for deoxyribose nucleic acid. Nature 171, 737–38. https://doi.org/10.1038/171737a0
30Werden, L.K., Sugii, N.C., Weisenberger, L., Keir, M.J., Koob, G., Zahawi, R.A., 2020. Ex situ conservation of threatened plant species in island biodiversity hotspots: a case study from Hawai‘i. Biol. Conserv. 243, 108435. https://doi.org/10.1016/j.biocon.2020.108435
65 Westbrook, J.W., Zhang, Q., Mandal, M.K., Jenkins, E.V., Barth, L.E., Jenkins, J.W., Grimwood, J., Schmutz, J., Holliday, J.A., 2019. Genomic selection analyses reveal tradeoff between chestnut blight tolerance and genome inheritance from American chestnut (Castanea dentata) in (C. dentata x C. mollissima) x C. dentata backcross populations. BioRxiv 690693. https://doi.org/10.1101/690693
74Whitehead, H., Rendell, L., 2014. The Cultural Lives of Whales and Dolphins. Chicago: University of Chicago Press.
76Wild, S., Hoppitt, W.J.E., Allen, S.J., Krützen, M., 2020. Integrating genetic, environmental, and social networks to reveal transmission pathways of a dolphin foraging innovation. Curr. Biol. 30, 3024-3030.e4. https://doi.org/10.1016/j.cub.2020.05.069
29Wilmut, I., Schnieke, A.E., McWhir, J., Kind, A.J., Campbell, K.H.S., 1997. Viable offspring derived from fetal and adult mammalian cells. Nature 385, 810–13. https://doi.org/10.1038/385810a0
Media Attributions
- Figure10-1 © Mike Cole is licensed under a CC BY (Attribution) license
- Figure10-2 © Supple et al. is licensed under a CC BY (Attribution) license
- Figure10-3 © Mariuswalter is licensed under a CC BY-SA (Attribution ShareAlike) license
- Figure10-4 © Edesi et al. is licensed under a CC BY (Attribution) license
- Figure10-5 © David Eickhoff is licensed under a CC BY (Attribution) license
- Figure10-6 © Jan Theodor de Bry is licensed under a Public Domain license
- USNM No. 30936 | SIA_000095_B44_F04_013 © United States National Museum Photographic Laboratory is licensed under a Public Domain license
- Figure10-9 © Gail Whistance is licensed under a CC BY-ND (Attribution NoDerivatives) license
- Figure10-10 © Self-portrait is licensed under a Public Domain license
- Table10-1a © NOAA Photo Library is licensed under a CC BY (Attribution) license
- Table10-1b © Sanjay Acharya is licensed under a CC BY-SA (Attribution ShareAlike) license
- Table10-1c © Albert Kok is licensed under a Public Domain license
- Table10-1d © Adamo is licensed under a CC BY (Attribution) license
- Table10-1e © Yathin S Krishnappa is licensed under a CC BY-SA (Attribution ShareAlike) license
- Figure10-11 © Shannon et al. is licensed under a CC BY (Attribution) license
- Figure10-12 © Brask et al. is licensed under a CC BY (Attribution) license
- Figure10-13 © Villegas-Ríos et al. is licensed under a CC BY (Attribution) license
- Figure10-14 © Abrahms et al. is licensed under a CC BY (Attribution) license
Beebe, W., 1934. Half Mile Down. San Diego, CA: Harcourt Brace.
Tunesi, L., 2017. Remembering the Bathysphere, a steel ball that changed the course of ocean exploration. Atlas Obscura, August 16, 2017. http://www.atlasobscura.com/articles/bathysphere.
Chakravarti, L.J., van Oppen, M.J.H., 2018. Experimental evolution in coral photosymbionts as a tool to increase thermal tolerance. Front. Mar. Sci. 5. https://doi.org/10.3389/fmars.2018.00227
Govenar, B., 2012. Energy transfer through food webs at hydrothermal vents: linking the lithosphere to the biosphere. Oceanography 25, 246–55.
Ebert, D.A., Papastamatiou, Y.P., Kajiura, S.M., Wetherbee, B.M., 2017. Etmopterus lailae sp. nov., a new lanternshark (Squaliformes: Etmopteridae) from the northwestern Hawaiian Islands. Zootaxa 4237, zootaxa.4237.2.10. https://doi.org/10.11646/zootaxa.4237.2.10
Monterey Bay Aquarium Research Institute. (2015). Autonomous underwater vehicles. Accessed November 30, 2015, https://www.mbari.org/at-sea/vehicles/autonomous-underwater-vehicles/.
A self-replicating material that is present in nearly all living organisms as the main constituent of chromosomes. It is the carrier of genetic information.
Watson, J.D., Crick, F.H.C., 1953. Molecular structure of nucleic acids: a structure for deoxyribose nucleic acid. Nature 171, 737–38. https://doi.org/10.1038/171737a0
Odum, H.T., Odum, E.P., 1955. Trophic structure and productivity of a windward coral reef community on Eniwetok Atoll. Ecol. Monogr. 25, 291–320. https://doi.org/10.2307/1943285
Elton, C.S. 1958. The Ecology of Invasions by Plants and Animals. North Yorkshire, UK: Methuen.
Glover, A.G., Smith, C.R., 2003. The deep-sea floor ecosystem: current status and prospects of anthropogenic change by the year 2025. Environ. Conserv. 30, 219–41. https://doi.org/10.1017/S0376892903000225
Auster, P.J., Hodge, B.C., McKee, M.P., Kraus, S.D., 2020. A scientific basis for designation of the Northeast Canyons and Seamounts Marine National Monument. Front. Mar. Sci. 7. https://doi.org/10.3389/fmars.2020.00566
Billett, D.S.M., Jones, D.O.B., Weaver, P.P.E., 2019. Improving environmental management practices in deep-sea mining. In R. Sharma (Ed.), Environmental Issues of Deep-Sea Mining: Impacts, Consequences and Policy Perspectives. Cham, Switzerland: Springer International, 403–46. https://doi.org/10.1007/978-3-030-12696-4_15
Da Ros, Z., Dell’Anno, A., Morato, T., Sweetman, A.K., Carreiro-Silva, M., Smith, C.J., Papadopoulou, N., Corinaldesi, C., Bianchelli, S., Gambi, C., Cimino, R., Snelgrove, P., Van Dover, C.L., Danovaro, R., 2019. The deep sea: the new frontier for ecological restoration. Mar. Policy 108, 103642. https://doi.org/10.1016/j.marpol.2019.103642
Jamieson, A.J., Malkocs, T., Piertney, S.B., Fujii, T., Zhang, Z., 2017. Bioaccumulation of persistent organic pollutants in the deepest ocean fauna. Nat. Ecol. Evol. 1, 0051. https://doi.org/10.1038/s41559-016-0051
Regalado, A., 2020. China’s BGI says it can sequence a genome for just $100. MIT Technology Review, February 26, 2020. https://www.technologyreview.com/2020/02/26/905658/china-bgi-100-dollar-genome/.
A genetic screening method for discovering novel plant and animal SNPs and performing genotyping studies.
SNP and SNV genotyping are techniques that analyze genomic sequence variations. These single-base substitutions are typically detected using real-time PCR, microarrays, or next-generation sequencing (NGS) techniques. A single nucleotide variant (SNV) is a variation of a single nucleotide in a population's genome, and a single nucleotide polymorphism is a singular mutation between different individuals in a study population.
Functional genomics focuses on the dynamic expression of gene products in a specific context, for example, at a specific developmental stage. The previous knowledge of gene function is utilized to attempt to develop a model linking gene to function.
A genome-wide association study is an approach that involves rapidly scanning markers across the complete sets of DNA, or genomes, of many case studies in an individual study group to find variation associated with a disease or particular feature. Once new genetic associations are identified, researchers can use the information to answer questions of source, develop better medicines, or even perform phylogenic studies.
A quantitative trait is a measurable phenotype that depends on the cumulative actions of many genes and the environment. These traits can vary among individuals, over a range, to produce a continuous distribution of phenotypes. Examples include height, weight, number of branches, or spots on fur.
A quantitative trait locus (QTL) is a region of DNA associated with a specific phenotype or trait that varies within a population. Typically, QTLs are associated with traits with continuous variance, such as height, rather than those of discrete variance, or incomplete dominance, like flower coloring or cat fur.
Supple, M.A., Bragg, J.G., Broadhurst, L.M., Nicotra, A.B., Byrne, M., Andrew, R.L., Widdup, A., Aitken, N.C., Borevitz, J.O., 2018. Landscape genomic prediction for restoration of a Eucalyptus foundation species under climate change. ELife 7, e31835. https://doi.org/10.7554/eLife.31835
Watsa, M., Erkenswick, G.A., Pomerantz, A., Prost, S., 2020. Portable sequencing as a teaching tool in conservation and biodiversity research. PLoS Biol. 18, e3000667. https://doi.org/10.1371/journal.pbio.3000667
Breed, M.F., Harrison, P.A., Blyth, C., Byrne, M., Gaget, V., Gellie, N.J.C., Groom, S.V.C., Hodgson, R., Mills, J.G., Prowse, T.A.A., Steane, D.A., Mohr, J.J., 2019. The potential of genomics for restoring ecosystems and biodiversity. Nat. Rev. Genet. 20, 615–28. https://doi.org/10.1038/s41576-019-0152-0
Marker-assisted selection (MAS) is the process of using morphological, biochemical, or DNA markers as indirect selection criteria for selecting agriculturally important traits in crop breeding. This process is used to improve the effectiveness or efficiency of selection for the traits of interest in breeding programs.
Acclimatization is the beneficial physiological adaptations that occur during repeated exposure to a hot environment. These physiological adaptations include: Increased sweating efficiency (earlier onset of sweating, greater sweat production, and reduced electrolyte loss in sweat).
Epigenetics is the study of how your behaviors and environment can cause changes that affect the way your genes work. Unlike genetic changes, epigenetic changes are reversible and do not change your DNA sequence, but they can change how your body reads a DNA sequence.
A single carbon chain on an organic molecule.
Tobi, E.W., Slieker, R.C., Luijk, R., Dekkers, K.F., Stein, A.D., Xu, K.M., Consortium, B.I.O. S., Slagboom, P.E., Zwet, E.W., van Lumey, L.H., Heijmans, B.T., 2018. DNA methylation as a mediator of the association between prenatal adversity and risk factors for metabolic disease in adulthood. Sci. Adv. 4, eaao4364. https://doi.org/10.1126/sciadv.aao4364
Julian, C.G., 2017. Epigenomics and human adaptation to high altitude. J. Appl. Physiol. 123, 1362–70. https://doi.org/10.1152/japplphysiol.00351.2017
Thorson, J.L.M., Smithson, M., Beck, D., Sadler-Riggleman, I., Nilsson, E., Dybdahl, M., Skinner, M.K., 2017. Epigenetics and adaptive phenotypic variation between habitats in an asexual snail. Sci. Rep. 7, 1–11. https://doi.org/10.1038/s41598-017-14673-6
The epigenome consists of chemical compounds that modify, or mark, the genome in a way that tells it what to do, where to do it, and when to do it. Epigenomes control expression more than what can be expressed; there exists a range within each individual in a population described by an epigenome.
Rey, O., Eizaguirre, C., Angers, B., Baltazar‐Soares, M., Sagonas, K., Prunier, J.G., Blanchet, S., 2020. Linking epigenetics and biological conservation: towards a conservation epigenetics perspective. Funct. Ecol. 34, 414–27. https://doi.org/10.1111/1365-2435.13429
Transgenic refers to an organism or cell whose genome has been altered by the introduction of one or more foreign DNA sequences from another species by artificial means. Transgenic organisms are generated in the laboratory for research purposes.
An area of research seeking to modify genes of living organisms to improve our understanding of gene function and develop ways to use it to treat genetic or acquired diseases.
Samuel, M.D., Liao, W., Atkinson, C.T., LaPointe, D.A., 2020. Facilitated adaptation for conservation—can gene editing save Hawaii’s endangered birds from climate driven avian malaria? Biol. Conserv. 241, 108390. https://doi.org/10.1016/j.biocon.2019.108390
Cells containing two complete sets of chromosomes, one from each parent.
A natural process and technology of genetic engineering that propagates a particular suite of genes throughout a population by altering the probability that a specific allele will be transmitted to offspring (instead of the Mendelian 50% probability)
Grognet, P., Lalucque, H., Malagnac, F., Silar, P., 2014. Genes that bias Mendelian segregation. PLoS Genet. 10, e1004387. https://doi.org/10.1371/journal.pgen.1004387
Leitschuh, C.M., Kanavy, D., Backus, G.A., Valdez, R.X., Serr, M., Pitts, E.A., Threadgill, D., Godwin, J., 2018. Developing gene drive technologies to eradicate invasive rodents from islands. J. Responsible Innovation 5, S121–S138. https://doi.org/10.1080/23299460.2017.1365232
Esvelt, K.M., Gemmell, N.J., 2017. Conservation demands safe gene drive. PLoS Biol. 15, e2003850. https://doi.org/10.1371/journal.pbio.2003850
Sudweeks, J., Hollingsworth, B., Blondel, D.V., Campbell, K.J., Dhole, S., Eisemann, J.D., Edwards, O., Godwin, J., Howald, G.R., Oh, K.P., Piaggio, A.J., Prowse, T.A.A., Ross, J. V., Saah, J.R., Shiels, A.B., Thomas, P.Q., Threadgill, D.W., Vella, M.R., Gould, F., Lloyd, A.L., 2019. Locally fixed alleles: a method to localize gene drive to island populations. Sci. Rep. 9, 15821. https://doi.org/10.1038/s41598-019-51994-0
Wilmut, I., Schnieke, A.E., McWhir, J., Kind, A.J., Campbell, K.H.S., 1997. Viable offspring derived from fetal and adult mammalian cells. Nature 385, 810–13. https://doi.org/10.1038/385810a0
The body's raw materials — cells from which all other cells with specialized functions are generated. Under the right conditions in the body or a laboratory, stem cells divide to form more cells called daughter cells. In plants these are meristematic cells located in a meristem; the largest and most important of which are the root and shoot.
A developmental process where a plant somatic cell can dedifferentiate to a totipotent, or in botany terms, an initial, egg or binucleic megasporangia cell that has the ability to give rise to an embryo under appropriate conditions. This new embryo can further develop into a whole plant.
A method of plant propagation using extremely small pieces of plant tissue taken from a carefully chosen and prepared mother plant. These are grown under laboratory conditions to produce new plants. It is widely used in commercial horticulture.
From Latin. "Ex" for out of, "Situ" (SY-too) for "its original place. The type of conservation efforts typified by seed banks, herbariums, zoos, and conservatories.
A process that conserves, cells, tissues, organelles, or any other biological constructs by storing them in very low temperatures. Fry in Futurama, but miniature.
Werden, L.K., Sugii, N.C., Weisenberger, L., Keir, M.J., Koob, G., Zahawi, R.A., 2020. Ex situ conservation of threatened plant species in island biodiversity hotspots: a case study from Hawai‘i. Biol. Conserv. 243, 108435. https://doi.org/10.1016/j.biocon.2020.108435
Borges, A.A., Pereira, A.F., 2019. Potential role of intraspecific and interspecific cloning in the conservation of wild mammals. Zygote 27, 111–17. https://doi.org/10.1017/S0967199419000170
Marshall, C., 2020. Beaver families win legal “right to remain.” BBC News, August 6, 2020. https://www.bbc.com/news/science-environment-53658375.
Marr, M.M., Brace, S., Schreve, D.C., Barnes, I., 2018. Identifying source populations for the reintroduction of the Eurasian beaver, Castor fiber L. 1758, into Britain: evidence from ancient DNA. Sci. Rep. 8, 2708. https://doi.org/10.1038/s41598-018-21173-8
San Diego Zoo, 2021. California condor (Gymnogyps californianus) fact sheet: population and conservation status. Last updated November 2, 2021, https://ielc.libguides.com/sdzg/factsheets/californiacondor/population.
Robinson, J.A., Bowie, R.C.K., Dudchenko, O., Aiden, E.L., Hendrickson, S.L., Steiner, C.C., Ryder, O.A., Mindell, D.P., Wall, J.D., 2021. Genome-wide diversity in the California condor tracks its prehistoric abundance and decline. Curr. Biol. 31, 2939-2946.e5. https://doi.org/10.1016/j.cub.2021.04.035
D’Elia, J., Haig, S.M., Mullins, T.D., Miller, M.P., 2016. Ancient DNA reveals substantial genetic diversity in the California Condor (Gymnogyps californianus) prior to a population bottleneck. The Condor 118, 703–14. https://doi.org/10.1650/CONDOR-16-35.1
A comprehensive, often large-scale, conservation effort focused on restoring sustainable biodiversity and ecosystem health by protecting core wild/wilderness areas, providing connectivity between such areas, and protecting or reintroducing apex predators and highly interactive species (keystone species).
Norris, K., Terry, A., Hansford, J.P., Turvey, S.T., 2020. Biodiversity conservation and the Earth System: mind the gap. Trends Ecol. Evol. 35, 919–26. https://doi.org/10.1016/j.tree.2020.06.010
Donlan, C., Berger, J., Bock, C.E., Bock, J.H., Burney, D.A., Estes, J.A., Foreman, D., Martin, P.S., Roemer, G.W., Smith, F.A., Soulé, M.E., Greene, H.W., 2006. Pleistocene rewilding: an optimistic agenda for twenty‐first century conservation. Am. Nat. 168, 660–81. https://doi.org/10.1086/508027
Communities that represent ecological restorative comparisons; similar species and environments. Restoration efforts can be modeled after the successful progress of the analog.
Griffiths, C.J., Jones, C.G., Hansen, D.M., Puttoo, M., Tatayah, R.V., Müller, C.B., Harris, S., 2010. The use of extant non-Indigenous tortoises as a restoration tool to replace extinct ecosystem engineers. Restor. Ecol. 18, 1–7. https://doi.org/10.1111/j.1526-100X.2009.00612.x
Hansen, D.M., Donlan, C.J., Griffiths, C.J., Campbell, K.J., 2010. Ecological history and latent conservation potential: large and giant tortoises as a model for taxon substitutions. Ecography 33, 272–84. https://doi.org/10.1111/j.1600-0587.2010.06305.x
A new concept: areas that form the best route between current climate types and where those climates will occur in the future under climate change.
Areas that remain relatively buffered from contemporary climate change over time and enable persistence of valued physical, ecological, and socio-cultural resources . The key attribute of refugia is their relative persistence, despite changes in the climate in the surrounding landscape.
Morelli, T.L., Barrows, C.W., Ramirez, A.R., Cartwright, J.M., Ackerly, D.D., Eaves, T.D., Ebersole, J.L., Krawchuk, M.A., Letcher, B.H., Mahalovich, M.F., Meigs, G.W., Michalak, J.L., Millar, C.I., Quiñones, R.M., Stralberg, D., Thorne, J.H., 2020. Climate-change refugia: biodiversity in the slow lane. Front. Ecol. Environ. 18, 228–34. https://doi.org/10.1002/fee.2189
McGuire, J.L., Lawler, J.J., McRae, B.H., Nuñez, T.A., Theobald, D.M., 2016. Achieving climate connectivity in a fragmented landscape. Proc. Natl. Acad. Sci. 113, 7195–200. https://doi.org/10.1073/pnas.1602817113
Parks, S.A., Carroll, C., Dobrowski, S.Z., Allred, B.W., 2020. Human land uses reduce climate connectivity across North America. Global Change Biol. 26, 2944–55. https://doi.org/10.1111/gcb.15009
Stralberg, D., Carroll, C., Nielsen, S.E., 2020. Toward a climate-informed North American protected areas network: incorporating climate-change refugia and corridors in conservation planning. Conserv. Lett. 13, e12712. https://doi.org/10.1111/conl.12712
Pedrono, M., Griffiths, O.L., Clausen, A., Smith, L.L., Griffiths, C.J., Wilmé, L., Burney, D.A., 2013. Using a surviving lineage of Madagascar’s vanished megafauna for ecological restoration. Biol. Conserv. 159, 501–6. https://doi.org/10.1016/j.biocon.2012.11.027
Gómez-Ruiz, P.A., Sáenz-Romero, C., Lindig-Cisneros, R., 2020. Early performance of two tropical dry forest species after assisted migration to pine–oak forests at different altitudes: strategic response to climate change. J. Forest. Res. 31, 1215–23. https://doi.org/10.1007/s11676-019-00973-2
Gómez-Mendoza, L., Arriaga, L., 2007. Modeling the effect of climate change on the distribution of oak and pine species of Mexico. Conserv. Biol. 21, 1545–55.
Galla, S.J., Moraga, R., Brown, L., Cleland, S., Hoeppner, M.P., Maloney, R.F., Richardson, A., Slater, L., Santure, A.W., Steeves, T.E., 2020. A comparison of pedigree, genetic and genomic estimates of relatedness for informing pairing decisions in two critically endangered birds: implications for conservation breeding programmes worldwide. Evol. Appl. 13, 991–1008. https://doi.org/10.1111/eva.12916
Johnson, W.E., Onorato, D.P., Roelke, M.E., Land, E.D., Cunningham, M., Belden, R.C., McBride, R., Jansen, D., Lotz, M., Shindle, D., Howard, J., Wildt, D.E., Penfold, L.M., Hostetler, J.A., Oli, M.K., O’Brien, S.J., 2010. Genetic restoration of the Florida panther. Science 329, 1641–45. https://doi.org/10.1126/science.1192891
Revive and Restore, 2022. The great passenger pigeon comeback. Accessed September 18, 2022, https://reviverestore.org/about-the-passenger-pigeon/.
Kasperbauer, T.J., 2017. Should we bring back the passenger pigeon? The ethics of de-extinction. Ethics Policy Environ. 20, 1–14. https://doi.org/10.1080/21550085.2017.1291831
Norström, A.V., Nyström, M., Jouffray, J.-B., Folke, C., Graham, N.A., Moberg, F., Olsson, P., Williams, G.J., 2016. Guiding coral reef futures in the Anthropocene. Front. Ecol. Environ. 14, 490–98. https://doi.org/10.1002/fee.1427
Baums, I.B., Baker, A.C., Davies, S.W., Grottoli, A.G., Kenkel, C.D., Kitchen, S.A., Kuffner, I.B., LaJeunesse, T.C., Matz, M.V., Miller, M.W., Parkinson, J.E., Shantz, A.A., 2019. Considerations for maximizing the adaptive potential of restored coral populations in the western Atlantic. Ecol. Appl. 29, e01978. https://doi.org/10.1002/eap.1978
Miller, M.W., Baums, I.B., Pausch, R.E., Bright, A.J., Cameron, C.M., Williams, D.E., Moffitt, Z.J., Woodley, C.M., 2018. Clonal structure and variable fertilization success in Florida Keys broadcast-spawning corals. Coral Reefs 37, 239–49. https://doi.org/10.1007/s00338-017-1651-0
Randall, C.J., Negri, A.P., Quigley, K.M., Foster, T., Ricardo, G.F., Webster, N.S., Bay, L.K., Harrison, P.L., Babcock, R.C., Heyward, A.J., 2020. Sexual production of corals for reef restoration in the Anthropocene. Mar. Ecol. Prog. Ser. 635, 203–32. https://doi.org/10.3354/meps13206
Rinkevich, B., 2019. Coral chimerism as an evolutionary rescue mechanism to mitigate global climate change impacts. Global Change Biol. 25, 1198–206. https://doi.org/10.1111/gcb.14576
Schoepf, V., Carrion, S.A., Pfeifer, S.M., Naugle, M., Dugal, L., Bruyn, J., McCulloch, M.T., 2019. Stress-resistant corals may not acclimatize to ocean warming but maintain heat tolerance under cooler temperatures. Nat. Commun. 10, 4031. https://doi.org/10.1038/s41467-019-12065-0
Selmoni, O., Lecellier, G., Magalon, H., Vigliola, L., Benzoni, F., Peignon, C., Joost, S., Berteaux-Lecellier, V., 2020. Seascape genomics reveals candidate molecular targets of heat stress adaptation in three coral species. BioRxiv, 2020.05.12.090050. https://doi.org/10.1101/2020.05.12.090050
Clark, I., Jones, S.S., Reganold, J.P., Sanguinet, K.A., Murphy, K.M., 2019. Agronomic performance of perennial grain genotypes in the Palouse region of the Pacific Northwest, USA. Front. Sustainable Food Syst. 3.
Quigley, K.M., Randall, C.J., van Oppen, M.J.H., Bay, L.K., 2020b. Assessing the role of historical temperature regime and algal symbionts on the heat tolerance of coral juveniles. Biol. Open 9. https://doi.org/10.1242/bio.047316
Westbrook, J.W., Zhang, Q., Mandal, M.K., Jenkins, E.V., Barth, L.E., Jenkins, J.W., Grimwood, J., Schmutz, J., Holliday, J.A., 2019. Genomic selection analyses reveal tradeoff between chestnut blight tolerance and genome inheritance from American chestnut (Castanea dentata) in (C. dentata x C. mollissima) x C. dentata backcross populations. BioRxiv 690693. https://doi.org/10.1101/690693
Quigley, K.M., Bay, L.K., van Oppen, M.J.H., 2020a. Genome-wide SNP analysis reveals an increase in adaptive genetic variation through selective breeding of coral. Mol. Ecol. 29, 2176–88. https://doi.org/10.1111/mec.15482
Powell, W.A., Newhouse, A.E., Coffey, V., 2019. Developing blight-tolerant American chestnut trees. Cold Spring Harbor Perspect. Biol. 11, a034587. https://doi.org/10.1101/cshperspect.a034587
Polygenic inheritance refers to the inheritance of a trait governed by more than one genes. In humans, eye color. In cats, fur. Generally, three or more genes govern the inheritance of polygenic traits. Multiple independent genes have an additive or similar effect on a single quantitative trait.
Cleves, P.A., Strader, M.E., Bay, L.K., Pringle, J.R., Matz, M.V., 2018. CRISPR/Cas9-mediated genome editing in a reef-building coral. Proc. Natl. Acad. Sci. 115, 5235–40. https://doi.org/10.1073/pnas.1722151115
Muñiz-Castillo, A.I., Rivera-Sosa, A., Chollett, I., Eakin, C.M., Andrade-Gómez, L., McField, M., Arias-González, J.E., 2019. Three decades of heat stress exposure in Caribbean coral reefs: a new regional delineation to enhance conservation. Sci. Rep. 9, 11013. https://doi.org/10.1038/s41598-019-47307-0
van Oppen, M.J.H., Oliver, J.K., Putnam, H.M., Gates, R.D., 2015. Building coral reef resilience through assisted evolution. Proc. Natl. Acad. Sci. 112, 2307–13. https://doi.org/10.1073/pnas.1422301112
Howells, E.J., Beltran, V.H., Larsen, N.W., Bay, L.K., Willis, B.L., van Oppen, M.J.H., 2012. Coral thermal tolerance shaped by local adaptation of photosymbionts. Nat. Clim. Change 2, 116–20. https://doi.org/10.1038/nclimate1330
van Oppen, M.J.H., Blackall, L.L., 2019. Coral microbiome dynamics, functions and design in a changing world. Nat. Rev. Microbiol. 17, 557–67. https://doi.org/10.1038/s41579-019-0223-4
Bilas, R.D., Bretman, A., Bennett, T., 2021. Friends, neighbours and enemies: an overview of the communal and social biology of plants. Plant Cell Environ. 44, 997–1013. https://doi.org/10.1111/pce.13965
A learning process theory which proposes that new behaviors can be acquired by observing and imitating others.
Whitehead, H., Rendell, L., 2014. The Cultural Lives of Whales and Dolphins. Chicago: University of Chicago Press.
Garland, E.C., Goldizen, A.W., Rekdahl, M.L., Constantine, R., Garrigue, C., Hauser, N.D., Poole, M.M., Robbins, J., Noad, M.J., 2011. Dynamic horizontal cultural transmission of humpback whale song at the ocean basin scale. Curr. Biol. 21, 687–91. https://doi.org/10.1016/j.cub.2011.03.019
Danchin, E., Nöbel, S., Pocheville, A., Dagaeff, A.-C., Demay, L., Alphand, M., Ranty-Roby, S., van Renssen, L., Monier, M., Gazagne, E., Allain, M., Isabel, G., 2018. Cultural flies: conformist social learning in fruitflies predicts long-lasting mate-choice traditions. Science 362, 1025–30. https://doi.org/10.1126/science.aat1590
Fiorito, G., Scotto, P., 1992. Observational learning in Octopus vulgaris. Science 256, 545–47. https://doi.org/10.1126/science.256.5056.545
Kelly, T.R., Kimball, M.G., Stansberry, K.R., Lattin, C.R., 2020. No, you go first: phenotype and social context affect house sparrow neophobia. Biol. Lett. 16, 20200286. https://doi.org/10.1098/rsbl.2020.0286
Allen, C.R.B., Brent, L.J.N., Motsentwa, T., Weiss, M.N., Croft, D.P., 2020. Importance of old bulls: leaders and followers in collective movements of all-male groups in African savannah elephants (Loxodonta africana). Sci. Rep. 10, 13996. https://doi.org/10.1038/s41598-020-70682-y
Wild, S., Hoppitt, W.J.E., Allen, S.J., Krützen, M., 2020. Integrating genetic, environmental, and social networks to reveal transmission pathways of a dolphin foraging innovation. Curr. Biol. 30, 3024-3030.e4. https://doi.org/10.1016/j.cub.2020.05.069
Garland, E.C., McGregor, P.K., 2020. Cultural transmission, evolution, and revolution in vocal displays: insights from bird and whale song. Front. Psychol. 11. https://doi.org/10.3389/fpsyg.2020.544929
Aikens, E.O., Kauffman, M.J., Merkle, J.A., Dwinnell, S.P.H., Fralick, G.L., Monteith, K.L., 2017. The greenscape shapes surfing of resource waves in a large migratory herbivore. Ecol. Lett. 20, 741–50. https://doi.org/10.1111/ele.12772
Jesmer, B.R., Merkle, J.A., Goheen, J.R., Aikens, E.O., Beck, J.L., Courtemanch, A.B., Hurley, M.A., McWhirter, D.E., Miyasaki, H.M., Monteith, K.L., Kauffman, M.J., 2018. Is ungulate migration culturally transmitted? Evidence of social learning from translocated animals. Science 361, 1023–25. https://doi.org/10.1126/science.aat0985
Kühl , H.S., Boesch, C., Kulik, L., Haas, F., Arandjelovic, M., Dieguez, P., Bocksberger, G., McElreath, M.B., Agbor, A., Angedakin, S., Ayimisin, E.A., Bailey, E., Barubiyo, D., Bessone, M., Brazzola, G., Chancellor, R., Cohen, H., Coupland, C., Danquah, E., Deschner, T., Dowd, D., Dunn, A, Egbe, E.E., Eshuis, H., Goedmakers, A, Granjon, A.1, Head, J., Hedwig, D., Hermans,V., Imong, I., Jeffery, K., Jones, S., Junker, J., Kadam, P., Kambere, M., Kambi, M., Kienast, I., Kujirakwinja, D., Langergraber, K., Lapuente, J., Larson, B., Lee, K. Kevin, Leinert, V., Llana, M., Maretti, G, Marrocol, S, Martin, R., Mbi, J.T., Meier, A.C., Morgan, B., Morgan, D., Mulindahabi, F., Murai, M., Neil, E, Niyigaba, P, Ormsby, L.J., Orume, R., Pacheco, L., Piel, A., Preece, J., Regnaut, S., Rundus, A, Sanz, C., van Schijndell, J., Sommer, Volker, V., Stewart, F., Tagg, N., Vendras, E, Vergnes, V.3, Welsh, A., Wessling, E.G., Willie, J., Wittig, R.M.Roman, Yuh, Y.G., Yurkiw, K., Zuberbühler, K., Kalan, A.K. 2019. Human impact erodes chimpanzee behavioral diversity. Science 363, 1453–55. https://doi.org/10.1126/science.aau4532
Crates, R., Langmore, N., Ranjard, L., Stojanovic, D., Rayner, L., Ingwersen, D., Heinsohn, R., 2021. Loss of vocal culture and fitness costs in a critically endangered songbird. Proc. R. Soc. B Biol. Sci. 288, 20210225. https://doi.org/10.1098/rspb.2021.0225
Shannon, G., Cordes, L.S., Slotow, R., Moss, C., McComb, K., 2022. Social disruption impairs predatory threat assessment in African elephants. Animals 12, 495. https://doi.org/10.3390/ani12040495
Evolutionarily Significant Units (ESU) are defined by NOAA Fisheries under their Endangered Species Act (ESA) status reviews. They are defined as a population that is substantially reproductively isolated from cospecific populations and represents an important component in the evolutionary legacy of the species.
Papale, E.B., Azzolin, M.A., Cascão, I., Gannier, A., Lammers, M.O., Martin, V.M., Oswald, J.N., Perez-Gil, M., Prieto, R., Silva, M.A., Torri, M., Giacoma, C., 2021. Dolphin whistles can be useful tools in identifying units of conservation. BMC Zool. 6, 22. https://doi.org/10.1186/s40850-021-00085-7
United Nations Environment Programme, 2018. Report of the CMS Workshop on Conservation Implications of Animal Culture and Social Complexity. Presented at the Third Meeting of the Sessional Committee of the CMS Scientific Council (ScC-SC3), Bonn, May 29 to June 1, 2018. https://www.cms.int/en/document/report-cms-workshop-conservation-implications-animal-culture-and-social-complexity.
Morrison, R.E., Groenenberg, M., Breuer, T., Manguette, M.L., Walsh, P.D., 2019. Hierarchical social modularity in gorillas. Proc. R. Soc. B Biol. Sci. 286, 20190681. https://doi.org/10.1098/rspb.2019.0681
Social network analysis (SNA) is the process of investigating social structures through the use of networks and graph theory.
Sah, P., Méndez, J.D., Bansal, S., 2019. A multi-species repository of social networks. Sci. Data 6, 44. https://doi.org/10.1038/s41597-019-0056-z
Shier, D.M., 2006. Effect of family support on the success of translocated black-tailed prairie dogs. Conserv. Biol. 20, 1780–90. https://doi.org/10.1111/j.1523-1739.2006.00512.x
Dunston, E.J., Abell, J., Doyle, R.E., Kirk, J., Hilley, V.B., Forsyth, A., Jenkins, E., Freire, R., 2017. An assessment of African lion Panthera leo sociality via social network analysis: prerelease monitoring for an ex situ reintroduction program. Curr. Zool. 63, 301–11. https://doi.org/10.1093/cz/zow012
Tripovich, J.S., Popovic, G., Elphinstone, A., Ingwersen, D., Johnson, G., Schmelitschek, E., Wilkin, D., Taylor, G., Pitcher, B.J., 2021. Born to be wild: evaluating the zoo-based regent honeyeater breed for release program to optimise individual success and conservation outcomes in the wild. Front. Conserv. Sci. 2. https://www.frontiersin.org/article/10.3389/fcosc.2021.669563
Shier, D.M., Owings, D.H., 2007. Effects of social learning on predator training and postrelease survival in juvenile black-tailed prairie dogs, Cynomys ludovicianus. Anim. Behav. 73, 567–77. https://doi.org/10.1016/j.anbehav.2006.09.009
US Fish and Wildlife Service, 2022. Whooping crane. Retrieved July 11, 2022, https://www.fws.gov/species/whooping-crane-grus-americana.
Abrahms, B., Teitelbaum, C.S., Mueller, T., Converse, S.J., 2021. Ontogenetic shifts from social to experiential learning drive avian migration timing. Nat. Commun. 12, 7326. https://doi.org/10.1038/s41467-021-27626-5
Teitelbaum, C.S., Converse, S.J., Fagan, W.F., Böhning-Gaese, K., O’Hara, R.B., Lacy, A.E., Mueller, T., 2016. Experience drives innovation of new migration patterns of whooping cranes in response to global change. Nat. Commun. 7, 12793. https://doi.org/10.1038/ncomms12793
Sorel, M.H., Zabel, R.W., Johnson, D.S., Wargo Rub, A.M., Converse, S.J., 2021. Estimating population-specific predation effects on Chinook salmon via data integration. J. Appl. Ecol. 58, 372–81. https://doi.org/10.1111/1365-2664.13772
Schakner, Z.A., Buhnerkempe, M.G., Tennis, M.J., Stansell, R.J., van der Leeuw, B.K., Lloyd-Smith, J.O., Blumstein, D.T., 2016. Epidemiological models to control the spread of information in marine mammals. Proc. R. Soc. B Biol. Sci. 283, 20162037. https://doi.org/10.1098/rspb.2016.2037
Tidwell, K.S., Carrothers, B.A., Blumstein, D.T., Schakner, Z.A., 2021. Steller sea lion (Eumetopias jubatus) response to non-lethal hazing at Bonneville Dam. Front. Conserv, Sci. 2. https://www.frontiersin.org/articles/10.3389/fcosc.2021.760866
Edelblutte, É., Krithivasan, R., Hayek, M.N., 2022. Animal agency in wildlife conservation and management. Conserv. Biol., e13853. https://doi.org/10.1111/cobi.13853
Simões-Lopes, P.C., Daura-Jorge, F.G., Cantor, M., 2016. Clues of cultural transmission in cooperative foraging between artisanal fishermen and bottlenose dolphins, Tursiops truncatus (Cetacea: Delphinidae). Zoologia (Curitiba) 33. https://doi.org/10.1590/S1984-4689zool-20160107
Boonman-Berson, S., Turnhout, E., Carolan, M., 2016. Common sensing: human-black bear cohabitation practices in Colorado. Geoforum 74, 192–201. https://doi.org/10.1016/j.geoforum.2016.06.010
Garshelis, D.L., Baruch-Mordo, S., Bryant, A., Gunther, K.A., Jerina, K., 2017. Is diversionary feeding an effective tool for reducing human–bear conflicts? Case studies from North America and Europe. Ursus 28, 31–55. https://doi.org/10.2192/URSU-D-16-00019.1
California Department of Fish and Wildlife, 2022. DNA evidence prompts revised response to Tahoe Keys bear incidents. February 24, 2022, https://wildlife.ca.gov/Conservation/Mammals/Black-Bear/Blog/dna-evidence-prompts-revised-response-to-tahoe-keys-bear-incidents.