Genetic Improvement in Cereal Crops and the Green Revolution
As the field of genetics matured, it became possible for breeders to develop improved crop varieties using Mendelian genetics, genetic maps, and markers. A breeder usually combines the desired genes from two varieties via crossing and then selects progeny containing desired traits. If four different useful genes are found in four different wheat varieties, using a step-by-step approach, a breeder could bring all four genes in the one preferred variety. However, a breeder does not create new genes; he or she only combines preexisting genes present within the genetic pool. Therefore, the biodiversity of any species determines the limits of classical breeding. This chapter summarizes the genetic improvements made in three main cereal crops—maize, wheat, and rice—using the classical breeding approach and their role in making the green revolution successful in the latter part of the twentieth century. In the process, various international agricultural research centers evolved under the Consultative Group for International Agriculture (CGIAR) umbrella, which is playing a critical role in achieving global food security.
6.1 The Story of Maize
Maize—more popularly known as corn—is the main staple crop of the Americas. Maize (Zea mays L.) is a member of the grass family Poaceae (Gramineae). Mexico is the center of the domestication and diversification of maize (1). Maize’s ancestor is a wild grass, Teosinte parviglumis (2).[1] Until 1492, people outside of South, Central, and North America were unaware of maize’s existence. Native Americans have been cultivating maize for ~7,000 years.
Maize is unique among the cereal crops because it is monoecious (it bears separate male and female inflorescences on the same plant). The main stem terminates in a tassel (male inflorescence), and the silk containing female flowers is on the stem. The natural structure of the maize plant favors cross-pollination. The pollen from the male flowers reaches the female flowers (of the mother plant or other nearby maize plants) via wind. Thus in maize, an equal possibility of self-pollination and cross-pollination exists. The pollen of different plants can reach a female flower, leading to the fertilization of the egg cell independent of one another. After successful pollination, the seeds on a cob are not uniform because each seed results from an independent pollination event. In nature, genes are exchanged continuously between maize plants, which results in new combinations.
6.1.1 Discovery of Hybrid Vigor and F1 Hybrids of Maize
In 1876, William Beal, a scientist at the Michigan Agricultural College, conducted crossings between maize varieties. To control pollination, Beal cut off the male flowers of the female parent (this process is known as detasseling) to ensure 100 percent cross-pollination from the chosen male parent and 100 percent hybrid progeny. Beal observed that the yields obtained from hybrids were higher than either of their parental varieties. In 1877, Charles Darwin also experimented with crossing maize varieties. He observed that the offspring resulting from outcrossing produced 25 percent larger cobs than from selfing and that the hybrids had a greater ability to tolerate chilling stress.
In 1908, George H. Shull and Edward East independently experimented and developed purebreds of maize. They noticed that after successive cycles of selfing, the maize plants’ size and their yields decreased. This phenomenon was named inbreeding depression (see figure 6.1). Like Beal and Darwin, Shull also noticed that F1 hybrids are taller and healthier than their parents and produce higher yields; he named this phenomenon hybrid vigor or heterosis (see figure 6.1). Shull further made a very useful observation that after three generations of inbreeding, maize behaves almost like a purebred that can be used for producing the F1 hybrid. Such an approach could ensure 100 percent human control over pollination and good yields year after year. However, Shull did not have enough resources to continue his work on F1 hybrids of maize. In 1913, Shull migrated to Germany and became the editor of the scientific journal Genetics. East, a Harvard University professor, was also not able to pursue his research on maize, but he encouraged a new generation of scientists. One of his students, Donald F. Jones, crossed the two purebreds of maize to create an F1 hybrid, and afterward, by crossing two different F1 hybrids, he successfully created double-cross hybrids (see figure 6.1). Jones observed that the yield of the double hybrids was higher than single hybrids. Therefore, the most successful corn crop was the double-cross hybrid, and it laid the foundation for the widespread use of double-cross hybrids in the US. The hybrid vigor of maize was only maintained if new seeds were purchased every year, which made the seed industry commercially viable.
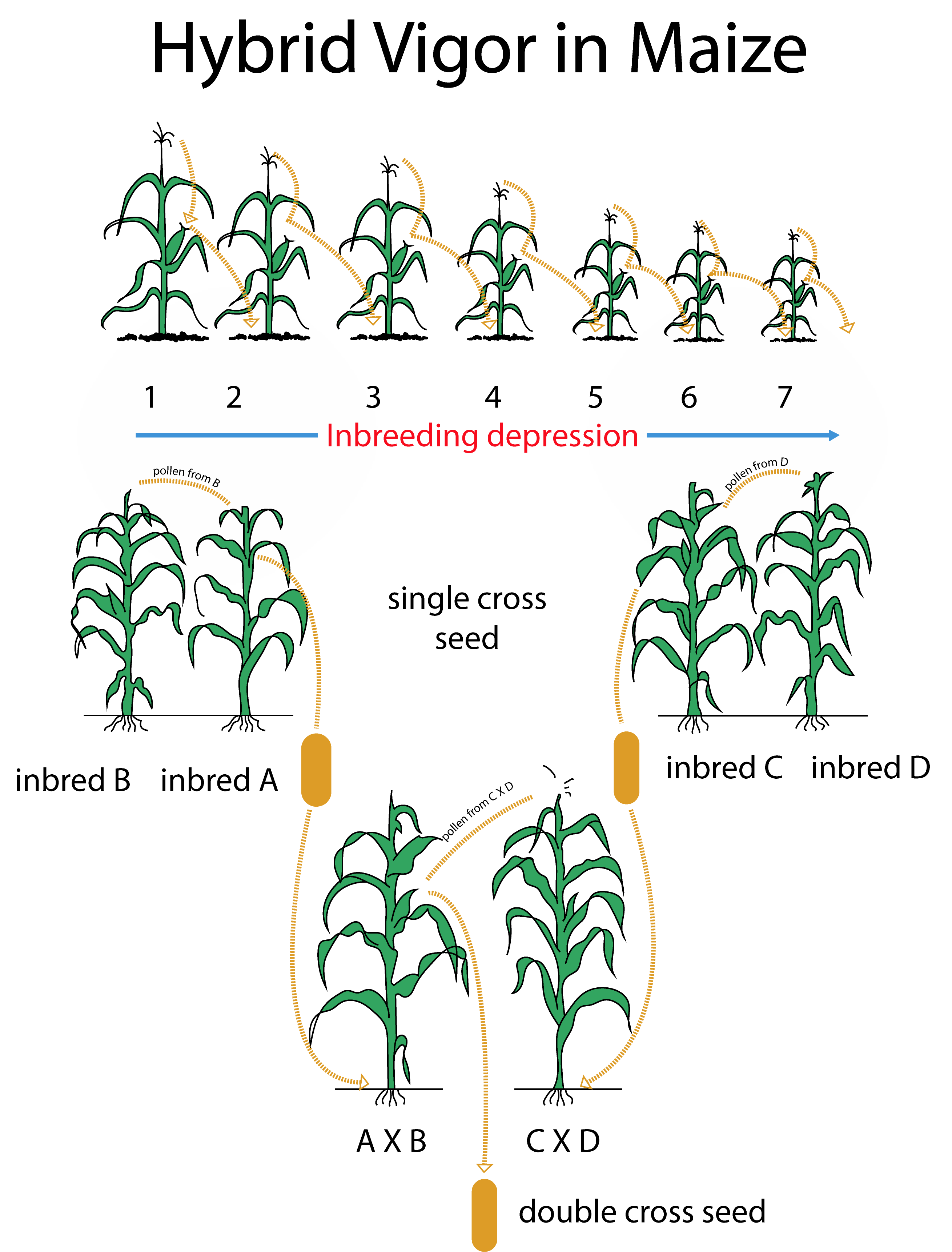
In the 1930s, the US Department of Agriculture helped farmers adopt F1 double-cross hybrid varieties of maize, leading to progressively higher yields. The first two hybrid varieties were grown side-by-side, and later to ensure 100 percent double hybrids, one row of pollen donor (male) was planted between every four rows of detasseled female plants. Gradually, most American farmers adopted this practice of maize cultivation. In 1933, less than 1 percent of total maize grown in the US was a double hybrid, but by 1944, it increased up to 56 percent. From 1930 to 1990, American scientists released about thirty-six improved hybrid varieties of maize, and farmers gladly adopted better varieties one after the other.
6.1.2 The US–Mexico Collaboration on Agriculture Research
In 1941, the vice president of the United States, Henry A. Wallace (1888–1965), traveled to Mexico to attend the inauguration ceremony of the newly elected president of Mexico, Manuel Ávila Camacho. Roosevelt and Wallace had also won elections in the US that same year. After attending Camacho’s ceremony, Wallace spent the first two days in the Bajio region with outgoing president Lázaro Cárdenas and newly elected agriculture secretary Marte Gómez. Wallace noticed that due to maize’s continuous cultivation, the Mexican soil had lost its fertility, and farming had become very unproductive. While the Iowa farmer spent ten hours to produce ten bushels of corn, it took a Mexican farmer 200 hours for the same. Wallace and his hosts discussed how the United States could help increase the productivity of Mexican farms. Wallace promised to help.
Upon his return to the US, Wallace contacted Raymond Fosdick, then the president of the Rockefeller Foundation, about providing US aid to Mexico and developing the appropriate project. Fosdick set up a three-member committee that consisted of maize geneticist Paul Mangelsdorf (Harvard University), agronomist Richard Bradfield (Cornell University), and plant pathologist Elvin Stakman (University of Minnesota) to evaluate a plan on agricultural cooperation between the US and Mexico. In 1942, these three experts recommended a pilot research program that led to the establishment of the Oficina de Estudios Especiales (OEE) program in 1943 with support from the Rockefeller Foundation and the Mexican government. The OEE’s mission was to train Mexican scientists across all disciplines to support the agricultural production of four major crops: maize, beans, wheat, and potatoes. The objective of establishing the Colegio de Postgraduados at Chapingo University in Texcoco, near Mexico City, was added in later years. Mangelsdorf supervised the maize research program, and Stakman was given the responsibility of directing the wheat breeding program.
6.1.3 Maize Germplasm Collection and Breeding from the 1950s to the 1960s
In 1943, a research project on maize was started under the supervision of Professor Paul Menzaldorf with the support of the Rockefeller Foundation. Menzaldorf’s team promoted F1 and double-cross hybrid varieties of maize developed by American scientists in Mexico. They also developed hybrids of local Mexican maize varieties. By 1947, ten new high-yielding double hybrids were released in Mexico under this program. In 1948, Mexico broke old records in maize production and became self-sufficient. By 1960, one-third of Mexico’s farms were cultivating high-yielding hybrids, and corn production had tripled. Menzaldorf’s team also began the collection of maize germplasm from Mexico and Central America. Since then, 28,000 maize varieties have been collected. This collection, representing almost all local maize varieties from eighty-eight countries, is stored at the Corn Germplasm Bank at the International Maize and Wheat Advancement Center (CIMMYT; https://www.cimmyt.org) located in Mexico City.
6.2 The Story of Wheat
Wheat is the second widely grown cereal in the world after maize. However, unlike maize, most of the wheat is consumed as food. Wheat (genus Triticum) has many diploid and polyploid species (see figure 6.2). The einkorn (T. monococcum L.), the oldest among domesticated wheat varieties, is a diploid species that contains the AA genome. A natural hybridization event between einkorn and a diploid weed goat grass with the BB genome gave rise to the emmer/durum wheat (T. turgidum). Therefore, emmer is tetraploid (AABB). Archaeological evidence suggests that the domestication of einkorn and emmer varieties occurred almost simultaneously (10,000–12,000 years ago) in the Fertile Crescent. Wild emmer (T. turgidum ssp. dicoccoides) is the progenitor of today’s tetraploid durum wheat (3).

A subsequent spontaneous natural hybridization event between emmer (AABB) and another species of wild grass, Aegilops tauschii (DD), gave rise to the hexaploid (AABBDD) spelt/dinkle or common bread wheat (T. aestivum) (3). Archaeological evidence suggests that spelt’s birthplace is the southeastern mountainous region of present-day Turkey. The polyploid durum and bread wheat produce larger seeds than the diploid species and their wild ancestors and also have a greater capacity to tolerate adverse conditions. Scientists estimate that around 7,000 years ago, the emmer wheat had spread outside its center of domestication and reached India, Central Asia, Egypt, and Europe. Emmer was the main cereal crop in Egypt at the time of the Pharaohs, while spelt was one of the major cereals of the Alemannians in southern Germany, Austria, and Switzerland between the twelfth and nineteenth centuries. Over time, many local varieties of wheat emerged in different regions due to the continued artificial selection of einkorn, emmer, and spelt in a variety of climates. Although these varieties show adaptations to local climates and may differ in morphological traits, most are subspecies of T. turgidum and T. aestivum. The wild einkorn and wild emmer have brittle rachis, while their domesticated descendants have nonbrittle rachis (see figure 6.3). This domestication trait caused the loss of shattering of mature seeds, and it became possible for the farmer to harvest the crop all at the same time.
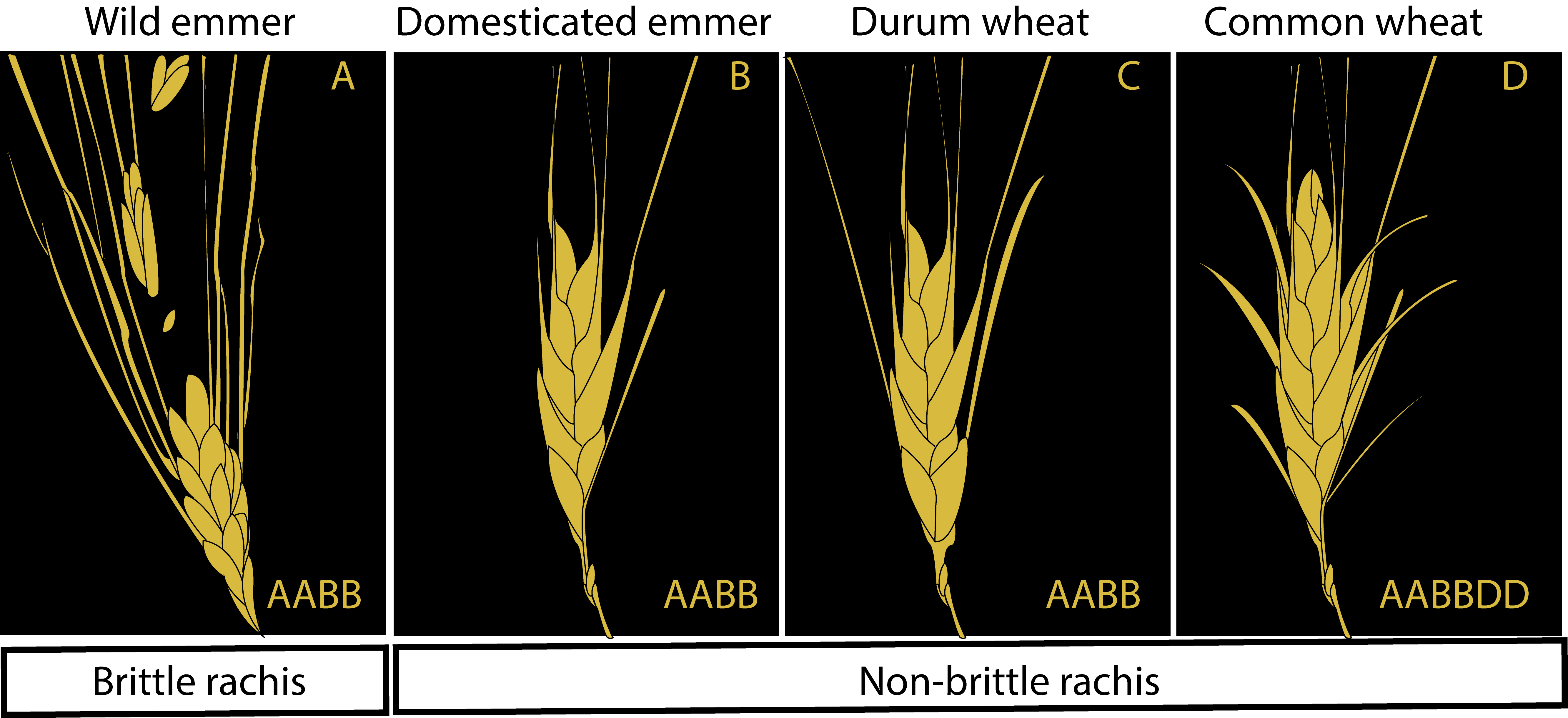
Spelt, emmer, and einkorn are hulled wheat species; their kernels cannot easily be extracted by threshing, and thus at harvest, the chaff remains at the kernels, and a special dehulling of the kernels is required in the mill to separate the chaff from the grain. A mutation in two genes, tenacious glume (Tg1) and q resulted in free-threshing wheat. Thus mutations in the Br1 loci on chromosomes 3A and 3B, Tg1 on 2D, and q on 5A had the most profound impacts on wheat’s domestication. In the last centuries, einkorn, emmer, and spelt were replaced by free-threshing durum and bread wheat. Durum (T. durum) is mainly used in the making of pasta and semolina. The common wheat (T. aestivum) is most suitable for bread, cookies, cakes, crackers, pastries, and noodles (it forms a spongier dough due to its higher gluten content and low gliadins/glutenins ratio). Ninety-five percent of wheat grown worldwide is common bread wheat, and the remaining is mostly durum. Recently, interest in ancient wheat species has been renewed for producing high-value baking goods due to their high nutrition content, and a market for catering to food-conscious elites is slowly growing.
6.2.1 The Beginning of Wheat Breeding Program in Mexico
In 1943, Stakman directed the OEE’s Cooperative Wheat Research and Production Program in Mexico. At that time, agricultural research in Mexico was almost at a standstill. There was no single agriculture scientist with a PhD and no active research program on agriculture in Mexico and South America. He recommended that Norman Ernest Borlaug (1914–2009), his former student, be brought to Mexico for a leading wheat breeding program. Borlaug had finished his PhD under the supervision of Stakman a year earlier, and he was working for DuPont as a scientist. In Mexico, George Harrar, a plant pathologist, oversaw the local unit. The three other scientists in his team (besides Norman Borlaug) were Edward Wellhausen, John Niederhauser, and William Colwell, all from the US.
Borlaug arrived in Mexico in 1944 to lead the International Wheat Improvement Program at El Batátan, Texcoco, outside of Mexico City. This was his first time traveling outside the US, and he didn’t know Spanish. He camped at a research center in the Yaqui Valley in Sonora, Mexico. In the 1930s, the governor of Sonora, Rodolfo Elias Calles, had set up that research station to help farmers. The station owned land for experimental research and some animals. The first director of this station, Edmundo Teboada, worked on some varieties of Mexican wheat and built a small wheat germplasm collection. But the research station did not have any modern farm equipment or any trained scientist.
There was no maintenance for this research station. So when Borlaug reached there, it was a mess. There was no electricity, and the windows and doors were broken. But Borlaug was not discouraged and managed to set up humble arrangements for his living quarters, and in the early morning, he started working like a farmer. Borlaug established connections with farmers in the Yaqui Valley, who were relatively prosperous and had a good irrigation system compared to other wheat-growing regions of Mexico. Borlaug borrowed tractors and other machinery for research from these farmers as needed. Between 1939 and 1941, the problem farmers faced in this area was primarily rust, a plant disease that would infect their wheat. So Borlaug initiated work on the development of a wheat that can fight rust. First, he selected the wheat varieties from Teboada’s collection, which showed some ability to resist rust. He then began a series of crossing and backcrossing experiments with wheat varieties to develop high-yielding, rust-resistant wheat (see figure 6.4).

6.2.2 Shuttle Breeding: Stem Rust-Resistant Wheat Varieties
Generally, it takes ten to twelve generations to combine useful desirable traits from two varieties of a crop by implementing classical breeding and selection methods. Thus at least ten years were needed for making a new breed of rust-resistant wheat. Borlaug thought that if two wheat crops could be grown per year, then a new variety of wheat could be made in five years. Fortunately for Borlaug, this opportunity was available in Mexico. In Sonora, wheat is sown in October and harvested in April. In the mountainous region of Toluca Valley in central Mexico, wheat is sown in April and May and harvested in October. Thus taking advantage of this, Borlaug began shuttle breeding. As soon as the wheat crop was harvested in Sonora (in April), Borlaug sent these seeds for sowing in Toluca. Similarly, after the crop harvest in Toluca (in October), new seeds were sent to Sonora. Borlaug’s shuttle breeding program was not well received by his seniors at the Rockefeller Foundation. Until that point, there was a general agreement that the wheat seeds need a resting period after harvest (or before sowing the following year). Stakman advised Borlaug not to waste time and resources. When Borlaug submitted his resignation in response, no one stopped him from using shuttle breeding.
Interestingly, the two locations (Yaqui and Toluca) used for shuttle breeding not only were 1,000 kilometers apart but also differed in latitude (by 10°) and elevation (by 2,000 meters; see figure 6.5). The Yaqui Valley is located at latitude 22° north and the Toluca Valley is located at latitude 12° north. Apart from this, the days of wheat cultivation in Yaqui Valley are shorter than in Toluca. As a result, the new wheat varieties developed by shuttle breeding became neutral to day length and regional climates. Those varieties could be grown on long and short days, on highland and valley, and at any elevation. These characteristics proved to be very helpful in spreading the improved varieties of wheat worldwide. The shuttle breeding experiment also reduced the time by half required for producing a variety using traditional breeding.

There was no agricultural extension program in Mexico to help Borlaug; he worked directly with the farmers. As he developed new breeds, he gave them directly to the local farmers for preliminary testing. If farmers had positive results, then the seeds of those varieties were sent to experts worldwide for detailed testing. In this way, he built collaborations with local farmers and scientists from all over the world. Between 1948 and 1950, Borlaug released eight new wheat varieties in Mexico, each of which seemed to be more resistant to rust. In addition to developing advanced varieties of wheat, Borlaug advised farmers on the right quantity of fertilizer to use and how to improve irrigation facilities. The best wheat yield in the Bajio region in Mexico without the use of fertilizers and irrigation yielded 1 ton/hectare. But after using sufficient fertilizers, irrigation, and weed management, these same wheat varieties yielded 7.5–8 tons/hectare.
In 1951–54, a rust epidemic devastated the wheat crop in North America due to the explosion of a highly pathogenic strain, 15b. By 1955, rust disease became a major challenge for American farmers. Under the pressure of this epidemic, many wheat nurseries were built around the world with the help of scientists from seventeen countries, and research on almost every kind of rust strain began. Also, the collection of wheat germplasm was undertaken afresh.
Since Borlaug already had several wheat varieties with rust resistance, he was assigned the responsibility of developing new varieties of American wheat and training other scientists. He built nurseries in Yaqui to test the wheat varieties’ ability to fight rust. He selected those varieties that had larger, more seeded panicles; were rust resistant; and could produce higher yields when a sufficient amount of fertilizer and irrigation was supplied. In this way, rust-resistant, high-yielding varieties were developed.
6.2.3 High-Yielding Dwarf Wheat
By 1950, the prevalent wheat varieties were six to eight feet long, and their thin stalk was weak, which often collapsed under the weight of their own grain—a trait called lodging. Moreover, the high-yielding varieties were more damaged by thunderstorms, as they grew even taller in response to synthetic fertilizer. Therefore, the breeders wanted to create a rust-resistant dwarf variety of wheat with a strong stem that can bear more weight. Borlaug had developed the rust-resistant, high-yielding wheat varieties, but he did not have any dwarf wheat in his collection. Thus the search for dwarf wheat was ongoing. Finally, S. Sisil Samon of the US Agricultural Research Service spotted a dwarf wheat variety, Norin-10, in Japan. He sent Norin-10 seeds to Professor Orville Fogle, who sent it to Borlaug.
In 1959, Borlaug crossed Norin-10 with some of his best North American varieties to create dwarf wheat varieties with a thicker, stronger stalk (e.g., Penjamo 620, Pittic 62, Gaines, Lerma Rojo 64, Siete Cerros, Sonora 64, and Super X). These high-yielding, rust-resistant dwarf varieties can stand the high winds, are amenable to machine harvests, and respond well to the application of fertilizers and irrigation. By 1963, 95 percent of Mexico’s wheat acreage was sown with these dwarf varieties. Thus a complete package was developed to increase wheat production, including improved seeds, synthetic fertilizer, pesticides and weedicides, irrigation, and machines. The implementation of this package in Mexico led to unexpected success in wheat production. In 1958, Mexico became self-sufficient, and in 1960, it started exporting wheat to other countries. The US also shared this success; from 1960 on, it became the leading exporter of wheat. These advancements in US agriculture laid the foundation for the green revolution, and millions of people worldwide were saved from starvation.
6.3 The Green Revolution
The Cold War began with the end of World War II. The world was divided into American and Soviet (USSR) camps. Apart from these two poles, there were many Asian, African, and Latin American countries that adopted a policy of remaining neutral to both camps. The nations were part of the Non-aligned Movement (NAM) in 1955, which was under the leadership of Jawaharlal Nehru (prime minister of India), Gamal Abdel Nasser (president of Egypt), and Josip Broz Tito (president of Yugoslavia). Most of the NAM countries had recently emerged from enslavement by former European empires, were economically weak, and were unable to produce enough grain for their populations. Due to continuous starvation and famine, there was a possibility of a “Red revolution” in these countries (like China and regions like Eastern Europe), which could have aligned with the USSR and alienated the US in the global politics; thus the US proposed a “green revolution” to neutralize the threat. The green revolution aimed at making developing countries self-sufficient in grain production. The hope was that if poor people could get enough food, then the need for a Red revolution would automatically disappear, and the US would not be isolated in the world.
At the heart of the green revolution were improved maize and wheat varieties developed by American scientists between 1930 and 1960 that yielded eight- to tenfold more grains when sufficient fertilizer, water, pesticides, and weedicides were applied. These modern packages of farming had been successfully implemented in Mexico and the US. The green revolution intended to repeat this experiment in Asia, Africa, and Latin America. The Rockefeller Foundation, Ford Foundation, and several other government and semigovernment organizations were given the responsibility to make the green revolution a success.
In 1960, Borlaug represented the Rockefeller Foundation in a Food and Agriculture Organization (FAO) committee. This committee reviewed the wheat-producing capacity of fifteen countries and concluded that except for India, Pakistan, Morocco, Bangladesh, Turkey, and Iran, most of the third world countries did not have agricultural scientists, and even the countries that had agriculture research programs were not capable of achieving food self-sufficiency. Thus the committee recommended training young breeders from developing countries in Mexico under Borlaug’s direction. The OEE project first proposed by Wallace eventually evolved into the International Maize and Wheat Improvement Center, a training center for breeders from all around the globe with the support of the Rockefeller and Ford Foundations and the Mexican government. Borlaug was appointed its director, where he served for the next thirty years.
In addition, Menzaldorf was given the responsibility of a maize breeding program for Latin America and Africa. Under the green revolution campaign, advanced varieties of maize developed by American scientists were introduced in Latin American countries. By 1980, high-yielding maize varieties were grown in 50 percent of South America’s arable land. However, the program was not as successful in introducing maize to Africa. Notably, the genetic improvement of rice was included in the green revolution’s agenda, as it was the major cereal crop of Asia.
Apart from improved seeds, institutional and infrastructural changes were needed for the green revolution to succeed. Farmers of developing countries used to save the seeds for the next sowing. They did not have the money to buy seeds, synthetic fertilizer, pesticides, and farming equipment. Thus the US entered into policy agreements with developing countries, including India, Pakistan, Bangladesh, Thailand, Indonesia, Egypt, and so on. The US provided seeds, fertilizer, technology, and training. Also, the US promised continued food aid to partner countries until they achieved self-sufficiency in food production. The host governments of developing countries had to build the necessary infrastructure and banking system to provide loans to farmers.
6.3.1 The Success of the Wheat Breeding Program in Asia
In the early 1960s, under Borlaug’s supervision, wheat breeding experiments began in India, Pakistan, and other Asian countries. In 1968, India harvested 16.6 million tons of wheat, for which an extra storage system was needed. Indira Gandhi, the then prime minister of India, used government schools as temporary godowns for grains during the summer months. Despite the drought in 1969 and 1970, 20 million tons of wheat were produced in India. Earlier, the average wheat production from 1963 to 1967 was 9–11 million tons. Pakistan also got about a 60 percent increase in wheat production. Between 1965 and 1970, Pakistan’s wheat yield increased from 4.6 million tons to 8.4 million tons and became self-sufficient in wheat production by 1968. Thus the green revolution had tremendous success in India and Pakistan and was met with similar success in Jordan, Lebanon, Turkey, and Indonesia. In many countries, the average life expectancy and living standards improved, and mortality rates declined significantly.
6.3.2 The Story of Rice
Unlike wheat and maize, very little research and breeding experiments were done on rice when it was included in the green revolution agenda. Thus in 1960, the Ford and Rockefeller Foundations, in collaboration with many international agencies, established the International Rice Research Institute[2] (IRRI; http://irri.org) in Los Baños in the Philippines. Robert Chandler (1907–91) was appointed as the first director of this institute and was responsible for establishing a rice germplasm collection and supervising the rice breeding program. Most of the research about rice’s origin and domestication, its genetic diversity, and its genetic improvement began after the 1960s.
However, before the improved rice varieties were made available, the farmers were encouraged to use synthetic fertilizer in rice fields, and an infrastructure for irrigation was developed. From 1950 to 1970, a 25 percent increase in rice production was achieved due to the availability of synthetic fertilization and irrigation. In the 1970s, rice production increased by five to six times due to improved rice germplasm; this helped the green revolution reach a peak because more than one-third of the world’s population is fed with rice. Even though there is more maize and wheat production in the world, maize only fulfills the need of 5 percent of the world’s population, and wheat fulfills 20 percent. In this sense, rice was the most important and representative crop of the green revolution. Here we summarize rice’s origin and genetics and the achievements of rice breeding in Asia and Africa.
6.3.2.1 The Origins of Rice
In nature, there are twenty-four species of grasses that are closely related to rice and are classified within the genus Oryza. These species are distributed in tropical and temperate regions in Asia, Africa, Australia, and South America. Some of these are diploid (2n = 24 chromosomes), and others are tetraploids (48 chromosomes). However, only two diploid species, Oryza sativa and Oryza glaberrima, were domesticated by humans. Interestingly, rice was independently domesticated in three different continents. O. sativa was domesticated in Asia and Australia. Archaeological and genetic evidence suggests that 9,000 to 11,000 years ago, the O. sativa subspecies japonica was first domesticated in the Yangtze River valley of China from the wild grass O. rufipogon. Another subspecies of rice, O. sativa indica, was independently domesticated 5,000 years ago in the Indo-Gangetic Plain of northern India from the wild grass O. nivara. In Australia, another subspecies of O. sativa was developed 2,000 years ago from O. rufipogon (4). The O. glaberrima was domesticated in Africa 3,500 years ago. O. glaberrima has evolved from the wild grass O. barthii in West Africa (in Mali), and then it was brought to North Africa and the Zanzibar islands (5). This rice arrived in the Americas with African slaves in the seventeenth century and has been grown in North Carolina (5). Among the two cultivated rice species, the O. sativa (Asian rice) is most widely grown globally and is the main staple for one-third of the human population.
6.3.2.2 The Diversity of Asian Rice
O. sativa is the most widely cultivated rice species in the world. Its japonica subspecies are grown in Southeast Asia, including China, Japan, Korea, Indonesia, Bali, Java, Sumatra, Vietnam, Cambodia, and so on. The japonica subspecies includes several thousand cultivars; some are adapted for the cold climate, while others flourish in the tropics. The varieties of japonica subspecies are broadly known as sticky/sushi rice and have a slightly sweet taste. The indica subspecies of O. sativa is mainly grown in the Indian subcontinent and South Asia. It also has tremendous diversity as well, including varieties adapted to rain-fed highlands and coastal regions. The cooked indica rice grains do not stick to one another and are known as basmati-type. Interestingly, the “sticky” feature is not found in the wild ancestors of rice and related wild species of the grass family. This trait, selected during domestication of the japonica subspecies, is caused by a mutation in the gene Waxi that blocks amylose production within the rice grain. Usually, two types of starch—amylose and amylopectin—are found in the rice grain, but only amylopectin is present in sticky rice. The glucose molecules in amylose form a simple linear structure, while in amylopectin, glucose molecules form branch chains that make amylopectin dissolve faster in hot water than amylose. Thus in sticky rice, the presence of amylopectin makes it sticky. In basmati rice, a high amount of amylose keeps the rice grain separated after cooking. In general, the ratio of amylose and amylopectin varies across cultivars, and accordingly, the different levels of stickiness and the variation in cooking time are observed (for this reason, two varieties of rice are not mixed for cooking).
Another interesting fact about rice is that rice grains are naturally red. The white color (or straw color; white is because of polishing) results from a mutation in a gene involved in the anthocyanin pigment’s[3] biosynthesis. For many years, it was a mystery to scientists why humans selected white over red for grain color. This feature was selected independently in both japonica and indica subspecies. It had been speculated that it was for its aesthetics or for some cultural belief. But in both China and India (the two birthplaces of Asian rice), red is considered auspicious, and there is no indication of any cultural preference for white. Genetic studies have recently revealed that the anthocyanin biosynthesis genes are linked to seed-shattering genes, and the loss of shattering also leads to the loss of the red pigment in rice. All the wild ancestors of rice have red seeds that spontaneously shatter upon maturation. This trait is useful for the survival of the wild grasses, but humans can’t harvest the crop at once. Thus a mutation that disrupted both seed shattering and anthocyanin biosynthesis gave rise to the rice plant whose straw-colored seeds did not fall upon maturation. Perhaps early humans picked a straw-colored mutant or collected seeds from more of such plants that favored its selection and propagation. Humans probably carried these plants forward in the process of domestication. Because color and seed-shattering genes are linked, red-colored seeds became less prevalent in the cultivated rice species.
The Asian rice O. sativa contains more than one gene for seed shattering and the biosynthesis of anthocyanin pigments. In domestication, two mutant (shattering) genes were selected in japonica that eliminated seed shattering and the red color. In indica, only one (shattering) gene was selected, and thus many cultivars have partial seed-shattering and red-colored seeds. Therefore, farmers in India harvest the paddy before it turns yellow and then dry it in the sun. It is believed that some of the traits of japonica were later introgressed in indica cultivars, and therefore, the modern varieties of indica rice are white.
Some varieties of both indica and japonica are found to contain long-grain rice. The comparison of 174 species of O. sativa found in different regions and forty species of its wild ancestor O. rufipogon has shown that the trait of grain length has been fixed in the process of domestication in tropical japonica, indica, and basmati. Basmati rice is the longest among them. The grains of other fragrant rice are also long. In contrast, japonica and Australian rice grown in temperate regions have relatively round and small rice grains (figure 6.6). Like all cereals, humans selected rice for large seeds and panicles and for its adaptation to various climates. This is how thousands of landraces of rice came into existence.
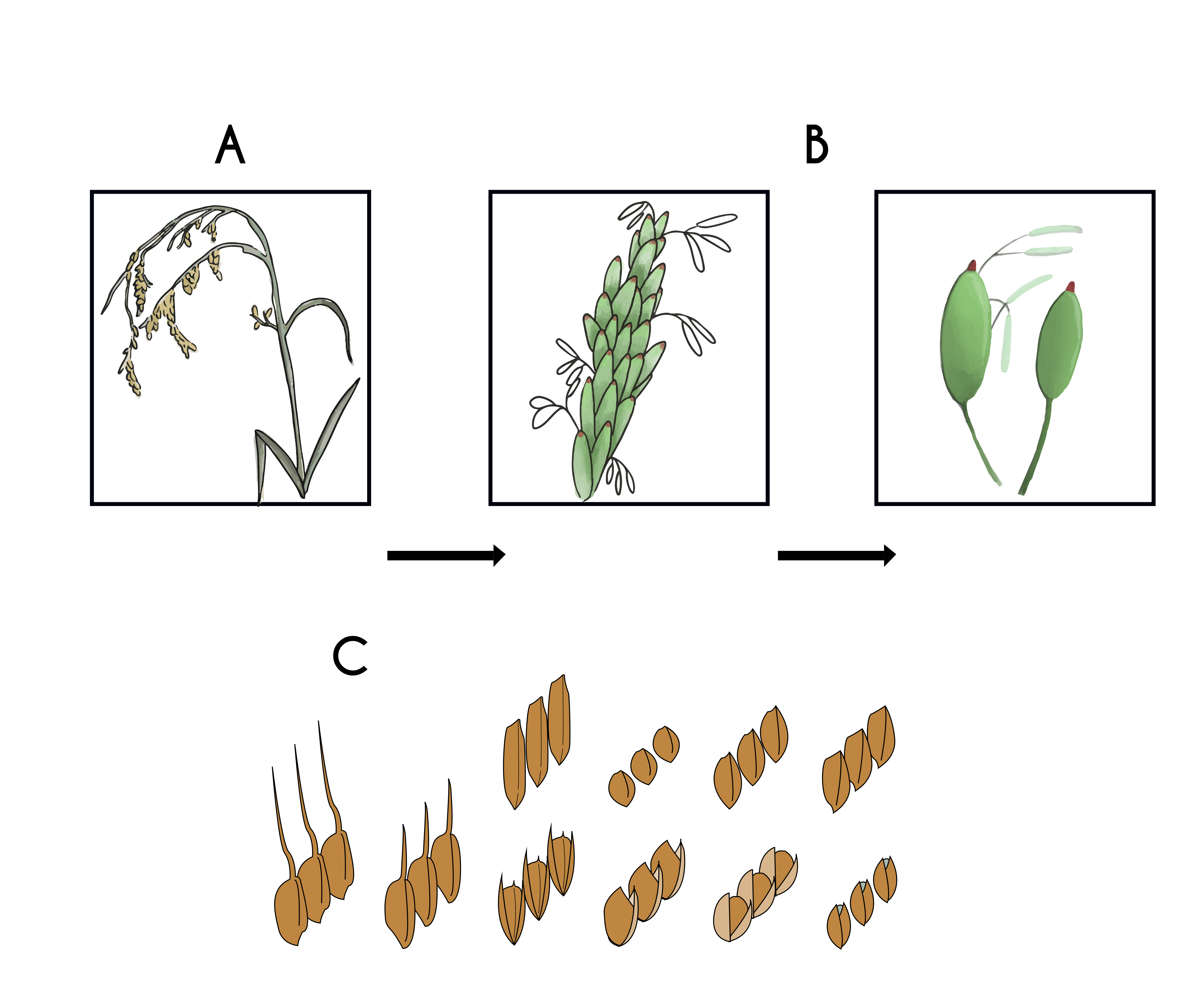
6.3.2.3 The Genetic Improvement of Rice at IRRI
After World War II, the shortage of grain and an increase in population caused widespread hunger in Asia. In 1949, the FAO established the International Rice Commission with the goal of increasing the yield of rice. The main obstacle in increasing the yield of the indica subspecies was the structure of this plant. Most indica cultivars were tall, had weak stems, and often collapsed on the ground when hit by storms or rains. Thus a strong dwarf stem was required for increasing rice productivity that can bear a heavy grain load.
In the 1950s, with FAO’s help, Indian scientists at Rice Research Center, Cuttack, developed rice ADT-27 and Mahsuri rice varieties by crossing Indian rice cultivars with a dwarf Taiwanese Taichung Native 1. In the 1960s, these hybrid rice varieties were grown on a large scale in India but received only a modest increase in yields. After the IRRI opened in the Philippines in the 1960s, Robert Chandler created a team of scientists to develop high-yielding rice varieties. Two members of this team, Peter Jenning and Akiro Tanaka, formulated the strategy for creating improved rice varieties. First, they carefully studied various indica and japonica cultivars. They concluded that if indica plants can be kept standing until maturity (they used bamboo sticks to support these plants), their yields are similar to those of dwarf japonica cultivars. Second, they observed that indica cultivars respond well to the application of synthetic fertilizer. Thus the main problem in the indica subspecies was the tall and weak stem. Jenning crossed dwarf Dee-geo-woo-gen (DGWG) rice variety (Taichung Native 1’s dwarf ancestor) with some indica and tropical japonica cultivars. By 1962, Jenning conducted thirty-nine crossings. He observed that first generation (F1) hybrid progeny was always tall and the F2 population segregated in the ratio of 3 tall:1 dwarf, suggesting that only one gene is responsible for stem’s tallness in Asian rice. Thus dwarfism could be easily introgressed into other rice varieties. Jenning also noticed that some of the dwarf plants matured one month earlier than the traditional rice varieties. The early maturation trait was of great importance because it could save thirty days of land use, fertilizer, and water, and in some regions, three rice crops could be grown a year instead of two. Afterward, Jenning tested seeds of early maturing dwarf plants in the nursery for resistance to blast disease and observed a partial resistance in one plant labeled as IR8-288-3 (IR8). The parents of IR8 were the dwarf DGWG and the Peta rice variety of Indonesia; IR8 was 120 centimeters tall; had a strong, thick stem capable of bearing a huge panicle full of seeds; and was not affected by the change in day length and altitude (thus it could be grown anywhere). Another scientist, S. K. D. Dutta, observed that IR8 yields are 9–10 tons/hectare when synthetic fertilizer, water, and weedicide are applied in adequate amounts.
In contrast, the average yield of traditional varieties was 1.2 tons/hectare. In that scenario, the IR8 emerged as a great alternative. It is said that when the news of IR8 reached Ferdinand Marcos (then the president of the Philippines), he immediately went to IRRI and ordered a large-scale multiplication of IR8 seeds. Marcos wanted to showcase this achievement in the next election. The US was also eager to hear some good news in Asia while engaged in the Vietnam War. So amid these pressures, IR8 was released in 1966 without further testing. It is said that 3,000 farmers from the Philippines’ remote islands came to IRRI to procure IR8 seeds. As expected, farmers got an increase of 5-10 times in yields by sowing IR8. With the help of IR8, starvation was temporarily avoided, and the foundation for making high-yielding rice varieties was laid.
The yield of IR8 was phenomenal, but it tasted chalky and was hard to chew. IR8 was also susceptible to various diseases, including bacterial blight and viral disease, and had only partial resistance to blast disease. Thus it required a heavy application of chemicals to keep the pests and pathogens away. In 1977, IRRI released IR36, which has a resistance to the blight and tungro viruses, and later IR64, which has an increased resistance to various diseases. Afterward, many rice varieties were developed by IRRI that showed increased resistance against many pathogens. These improved rice varieties were distributed across many rice-growing developing countries using governmental extension centers. Although the arms race between rice and its various pathogens continues, there has been no major famine due to rice shortage. The scientists at IRRI and in several other laboratories around the world are still breeding new rice varieties that can be more tolerant to abiotic stress and can withstand changing climates.
6.3.2.4 Yuan Longping’s Hybrid Rice Varieties
In wild ancestors of cultivated rice (O. rufipogon or O. barthii), self- and cross-pollination occur. In contrast, domesticated rice species O. sativa and O. glaberrima favor selfing over cross-pollination. Thus unlike in corn, the phenomenon of hybrid vigor is not easily observed in rice. In 1964, a Chinese scientist, Yuan Longping, decided to change the selfing nature of cultivated rice to make high-yielding hybrids rice varieties. When Longping began his research, the predominant thinking in the field was that hybrid vigor could not be applied to naturally self-fertilizing species such as rice, but he was convinced otherwise. After nine years of hard work, he developed three varieties of rice: the first breed was a male-sterile line incapable of making pollen; the second was a maintainer line that served as a source of pollen, and the third was a restorer line that can rescue a male-sterile line from sterility. Thus a cross between male-sterile and restorer lines produces completely fertile progeny. He demonstrated that hybrid progeny obtained by crossing the male-sterile line with the maintainer line shows hybrid vigor resulting in a significant increase in the grain yield. Generally, under favorable conditions, the yield of the most popular rice varieties is 5–6 metric tons/hectare. However, under similar growing conditions, hybrid rice released by Longping in 1974 gave an average yield of 7.2 metric tons/hectare (a 20 percent increase). Since 1976, this hybrid rice has been grown in China and provides food for an additional 70 million people. Since 1994, hybrid rice varieties have been sown in India, the Philippines, Bangladesh, and Indonesia. However, Longping continued his research on increasing grain yields. In 1997, yields of hybrid rice progeny were 10 metric tons/hectare, and by 2004, it reached 12 metric tons/hectare.
Professor Yuan Longping, the father of the hybrid rice, was awarded the UNESCO Science Prize in 1987; the World Food Prize,[4] with Monty Jones (who developed NERICA rice varieties for Africa and is discussed in the following section), in 2004; and the Wolf Prize in 2004 for his contribution to agriculture. In 2007, he was elected a foreign member of the US National Science Academy.
6.3.2.5 NERICA: The New Rice for Africa
African rice (O. glaberrima) was developed 3,500 years ago in West Africa from the wild O. barthii in the delta region of the Niger River (present-day Mali). African rice plants are more elongated and have weaker stems than Asian rice. These plants are relatively more susceptible to lodging and cannot bear the load of a heavy panicle. Also, seed shattering in African rice results in a significant loss in yields, as this trait was not selected against during domestication of African rice. However, this rice has the ability to survive in the harsh and challenging environment of Africa; it has a natural resistance against the various pests, parasites, and pathogens prevalent in its environment. Like Asian rice, African rice consists of many cultivars that represent a rich biodiversity, and many useful genes are hidden within these varieties. For example, the O. glaberrima CG-14 variety has a natural ability to tolerate drought, grows faster than weeds, and thrives on marginal land (e.g., deficient in phosphorus or acidic soil). Although the input costs for growing this crop are less, it has less productivity, and thus it is not widely grown.
For a long time, the Asian rice O. sativa has been grown in Africa on a large scale, as it is highly productive. Asian rice was introduced about 450 years ago in many countries of Africa. But Asian rice does not possess the ability to cope with Africa’s environment. Its yield decreases in drought conditions, and it cannot protect itself from parasites, pests, pathogens, and insects. The input cost for growing Asian rice is very high, as farmers use large amounts of synthetic fertilizer, pesticides, weedicides, and so on. Thus the sustainable cultivation of Asian rice in Africa poses a challenge. Unfortunately, the useful traits of African and Asian rice cannot be combined using classical breeding methods, as crossings between O. sativa and O. glaberrima yield sterile hybrids. Thus the green revolution failed in improving the productivity of African rice or the resilience of Asian rice for the African environment using classical breeding methods.
However, in the 1970s, African scientist Monty Jones, working at the West Africa Rice Advancement Institute (WARDA), collected 1,500 varieties of O. glaberrima and developed genetically improved varieties of African rice that show high resilience in the African environment. In the 1990s, when the technology of tissue culture became easier to apply on rice, Jones’s team created hybrid rice cells by fusing Asian and African rice cells to create hybrid plants from them. These plants were not sterile and can be grown from seeds. This new hybrid variety, called NERICA (New Rice for Africa), has a yield that is five times that of African rice cultivars, is resistant to various biotic and abiotic stress conditions prevalent in Africa, and has fewer input costs. NERICA also matures within three months, while its ancestral African varieties take six months. Thus Jones and his team could combine the useful characteristics of two rice species using advanced in vitro technology of plant tissue culture and surpassed the limits of traditional plant breeding. Farmers who grow NERICA also save three months of land use and labor cost and grow extra short-duration crops such as vegetables.
NERICA was Africa’s first successful genetically improved crop. For this work, the United Nations awarded the 2004 World Food Prize to Jones. In the wake of this success, Time magazine included Jones in the list of the most influential people in the world in 2007 (6). Jones’s method was subsequently applied to generate 3,000 new varieties of NERICA, which are being grown in African countries like Benin, Ivory Coast, Gambia, Nigeria, Mali, Guyana, Togo, and so on, and many of these countries have become rice exporters.
6.4 The Green Revolution’s Achievements
The green revolution was successful in increasing grain production in Asia, Latin America, and Africa. It saved the lives of millions from hunger and starvation. It can be said that the green revolution played an important role in maintaining peace in the world.
It also laid the foundation of mutual cooperation among various international institutes and scientists that lasted beyond the duration and need of the green revolution. Due to the political understanding, various international institutions and government machinery, private foundations, and banks worked together to foster scientific progress and ensure the food security of the world.
The green revolution was most successful in Asia because Asian countries already had the infrastructure and roads and had already developed a capitalist market system. During this period, many Asian countries—including India, Pakistan, Turkey, China, Sri Lanka, Indonesia, Malaysia, Vietnam, and Cambodia—became not only self-sufficient in food production but exporters of grains. For example, Pakistan achieved self-sufficiency in grain production in 1969; India followed this success in 1974, which was not expected by the world at that time. Similarly, when the IR8 rice variety was first released in the Philippines, it changed the country from an importer of rice to an exporter within just three years.
Overall, due to the increase in the yield of rice and wheat, food grains became cheaper, and the conditions of starvation and famine in developing countries could be avoided. The increase in grain yield per hectare prevented the expansion of cultivated land and helped protect the forests. According to one estimate, in the absence of the green revolution, by the year 2000, the world grain production would have been reduced by 20 percent, an additional 2–2.5 million hectare of agricultural land would have been needed to satisfy the food requirement of the current world population, and the cost of grain would be 30 percent higher.
Research in international centers also benefited the US, and the country’s domestic agriculture also increased. Between 1960 and 2000, in developed countries, the yield of wheat increased by 208 percent, rice by 109 percent, maize by 157 percent, potato by 78 percent, and cassava by 36 percent. Overall, the standard of living in developed countries was also improved due to the availability of cheaper food grains. Furthermore, the green revolution brought prosperity to the lives of many farmers and helped agriculture-related businesses flourish. US companies made tremendous profits by selling fertilizers, pesticides, weedicides, and agricultural equipment in the international market, and the green revolution helped recover the US from the post–World War II recession.
The green revolution failed in Africa due to various reasons, including the late inclusion of Africa on the agenda and the promotion of maize[5] instead of African crops. Furthermore, African countries lacked the agricultural infrastructure and trained professionals for disseminating adequate training to farmers about the use of fertilizers, pesticides, machines, and so on. Consequently, maize varieties that were successful in the US and Mexico were not successful in Africa. By 1998, while 82 percent of agricultural land in Asian countries, including China, India, and Pakistan, was growing genetically improved varieties of cereal crops, in Africa, only 27 percent of the area had these crops. However, many useful lessons were learned from the first phase of the green revolution (1965–85). The biggest gift of this period has been to lay the foundation for cooperation between agricultural scientists around the world. Later, scientists and policymakers succeeded in solving Africa’s problems in the second phase of the green revolution (1985–2000). During this period, fourteen other CGIAR research centers were opened, each focusing on the prime regional crop (eleven major crops, including potatoes, pulses, cassava, peanuts, beans, millets, and sorghums). The goal of each of these centers, which are spread around the world, is to conserve and improve the germplasm of local crops. From time to time, these international centers release advanced varieties of one or more crops to farmers. Studies show that worldwide production of wheat, rice, and maize has increased by 1 percent, 0.8 percent, and 0.7 percent, respectively, due to germplasm improvement during the second phase of the green revolution. Similarly, yields of minor grains like sorghum and millet have increased about 0.5 percent annually since 2000. International cooperation among scientists and various governmental and private agencies was also beneficial to many African countries, and after 1980, efforts to improve the local African crops, including rice, yam, cassava, and so on, began. Even after the end of the green revolution, these efforts are continuing.
Despite a nearly threefold increase in the world’s population in the last fifty years, no major famine occurred. Even today, the world’s population is producing more food than is needed. The green revolution played an important role in achieving global food security.
6.5 The Limits of the Green Revolution
The green revolution stood on the maximum exploitation of resources. Therefore, after about fifty to seventy years of intensive grain cultivation, soil fertility has decreased, groundwater levels have fallen significantly, and the overflow of agrochemicals led to the pollution of various water bodies. But by 1980, wheat production showed a slow decline by about 1.5 percent annually, and so far, it has fallen more than 20 percent than the 1970s and ’80s. On average, there has been a one-third decline in wheat production per hectare since the green revolution despite the continuous use of synthetic fertilizer, irrigation, and other mandated agrochemicals required to keep a check on pathogens and weeds. The high-yielding varieties of wheat, rice, and maize absorb large quantities of nutrients from the soil. The synthetic fertilizers replenish three major elements—nitrogen, phosphorus, and potassium (a.k.a. NPK)—but do not replace micronutrients and soil organic matter. Thus in many places, the soil has lost its normal texture, and it has turned almost sand-like. Recuperating the health of the soil is necessary, which cannot be done by continuing the green revolution’s model. The industrial method of farming needs to be reviewed and improved.
The goal of the green revolution was to achieve an increase in global food production, and during its implementation, the health of the ecosystem was not taken into consideration. The excessive use of pesticides, fertilizers, and weedicides polluted the groundwater, water bodies, and the air. Today, the entire food chain has become contaminated, and cancer and other diseases have increased in farmers and consumers. In some countries, positive efforts have been made in this direction. Today, breeders around the world are trying to develop varieties of crops that require less synthetic fertilizer, less irrigation, and fewer pesticides to grow. Recently, a super green rice variety has been released in the Philippines, which can maintain its productivity with less fertilizer and water use.
Before the green revolution, the staple diet of most Asian countries consisted of a wide variety of coarse cereals such as sorghums, millets, pulses, starchy tubers, and roots, which provided essential nutrients such as iron, calcium, vitamins, and micronutrients. There was no help for farmers growing other crops in the green revolution scheme, so these crops’ production decreased. The majority of the population’s diet has become homogenous, mainly consisting of rice, wheat, and maize. Therefore, malnutrition and devastating lifestyle diseases like diabetes increased despite getting plenty of calories.
The varieties of crops that are being grown today are few, and thousands of local varieties of crops have disappeared. Farmers no longer grow them, and thus our food sources are very limited and the diversity of crops has decreased significantly. The outbreak of several diseases has also revealed the importance of biodiversity and the limits of monocropping, which was encouraged by the green revolution. Thus despite achieving a high productivity of cereal crops, the green revolution package was not sustainable.
6.6 The Life of Norman Borlaug
Norman Borlaug, the father of the green revolution, was born on a farm near Cresco, Iowa, to Henry and Clara Borlaug.[6] He worked on the 106-acre family farm raising corn, oats, cattle, pigs, and chickens from age seven to nineteen. After completing his primary and secondary education in Cresco, Borlaug enrolled in the University of Minnesota and received a bachelor’s degree in 1937, a master’s degree in 1939, and a PhD in 1942. From 1942 to 1944, he was a microbiologist for DuPont, where he worked on industrial and agricultural bactericides, fungicides, and preservatives. In 1944, he accepted a position as a geneticist and plant pathologist assigned the task of wheat research and production program in Mexico, supported by the Rockefeller Foundation and the Mexican government. As described earlier, in that position, Borlaug successfully developed high-yielding, dwarf, disease-resistant wheat varieties.
He built collaborations with local farmers and scientists from all over the world. Borlaug had a deep connection with the farmers, and farming was no stranger to him. Childhood experiences had been with Barlog throughout his life. He was always trying to end hunger in the world. Equally important to his training with Stakman were his life experiences, which helped him sustain hardships for nearly two decades in Mexico’s difficult conditions. When the Rockefeller and Ford Foundations cooperated with the Mexican government to establish CIMMYT, Borlaug was its first director.
He is credited with saving more than a billion people around the world from starvation. In 1970, Borlaug received the Nobel Peace Prize. For the first time, a scientist received this award. Shortly after receiving the Nobel Prize, Borlaug established the World Food Prize to honor other scientists and breeders who made outstanding contributions.
Borlaug was also awarded the US Congressional Gold Medal, US Presidential Medal of Freedom, Pakistan’s Sitara-e-Imtiaz (1968), and India’s second-highest civilian honor, Padma Vibhushan (2006). Overall, Borlaug was a member of the Agricultural Sciences Academies of eleven countries and received more than sixty honorary doctoral degrees and around fifty other awards. Borlaug also received extensive recognition from universities and organizations in six countries: Canada, India, Mexico, Norway, Pakistan, and the United States.
References
Hastorf, C. A. (2009). Rio Balsas most likely region for maize domestication. Proc. Natl. Acad. Sci. USA, 106, 4957–58. (↵ Return)
Beadle, G. W. (1939). Teosinte and the origin of maize. Journal of Heredity, 30, 245–47. (↵ Return)
Faris, J. D. (2014). Wheat domestication: Key to agricultural revolutions past and future. In R. Tuberosa, A. Graner, & E. Frison (Eds.), Genomics of plant genetic resources: Vol. 1, Managing, sequencing, and mining genetic resources (pp. 439–64). Springer. (↵ Return 1) (↵ Return 2)
Kovach, M. J., Sweeney, M. T., & McCouch, S. R. (2007). New insights into the history of rice domestication. Trends in Genetics, 23, 578–87. https://doi.org/10.1016/j.tig.2007.08.012 (↵ Return)
Carney, J. A. (2002). Black rice: The African origins of rice cultivation in the Americas (1st ed.). Harvard University Press. (↵ Return 1) (↵ Return 2)
Sachs, J. (2007, May 3). Monty Jones. Time. http://content.time.com/time/specials/2007/time100/article/0,28804,1595326_1595329_1616316,00.html (↵ Return)
Further Readings
Borlaug, N. E. (2007). Sixty-two years of fighting hunger: Personal recollections. Euphytica, 157, 287–97. https://doi.org/10.1007/s10681-007-9480-9
Brown, L. R. (1970). Seeds of change: The green revolution and development in the 1970s. Praeger.
Coffman, R. (2010). Mentored by greatness. Indo-US science & technology forum. World Food Prize. https://www.worldfoodprize.org/index.cfm/88533/18129/mentored_by_greatness
Myrdal, G. (1970). Agriculture. In The challenge of world poverty: A world anti-poverty program in outline (chap. 4, pp. 78–138). Pantheon.
- For the story of maize’s domestication and origin, see the video “Popped secret: The mysterious origin of corn—HHMI BioInteractive Video” on YouTube: https://www.youtube.com/watch?v=mBuYUb_mFXA&t=51s. ↵
- Borlaug and Chandler discuss the origins of the International Rice Research Institute in a discussion filmed in 1994, now available as a multipart series on YouTube: Part 1: https://www.youtube.com/watch?v=17ySNZo3AMs Part 2: https://www.youtube.com/watch?v=TW8hpPi0rqI Part 3: https://www.youtube.com/watch?v=mEjQbo-2nZQ Part 4: https://www.youtube.com/watch?v=rQon8EfQblY Part 5: https://www.youtube.com/watch?v=jhqQwfc0-No Part 6: https://www.youtube.com/watch?v=jtuB2cTEfTU ↵
- Anthocyanin pigments are also found in many flowers, fruits, and vegetables and are beneficial for health. ↵
- “A world-brand name: Yuan Longping, the father of hybrid rice,” World Food Prize, 2007, https://www.worldfoodprize.org/index.cfm/87428/40007/a_worldbrand_name_yuan_longping_the_father_of_hybrid_rice. ↵
- Maize cultivation requires a lot of fertilizer and irrigation, but most areas of Africa lack water, and large dams could not be built for irrigation. ↵
- For Norman Borlaug’s biography, see https://achievement.org/achiever/norman-e-borlaug and http://nobelprize.org/nobel_prizes/peace/laureates/1970/borlaug-bio.html. ↵