The Early History of Genetics
No-one can say why the same peculiarity in different individuals…is sometimes inherited and sometimes not so: why the child often reverts in certain characters to its grandfather, or other much more remote ancestor; why a peculiarity is often transmitted from one sex to both sexes, or to one sex alone, more commonly but not exclusively to the like sex.
—Charles Darwin, On the Origin of Species
Our ancestors started agriculture with a certain sense that traits are inherited from parents to progeny. Centuries of breeding domestic animals and plants showed that useful traits could be accentuated by controlled mating, and as we have discussed in chapter 2, domestication and artificial selection gave rise to most modern crops. However, there was no rational way to predict the outcome of a cross between two parents or understanding how and why certain traits show up while others remain hidden (see figure 5.1). Until the nineteenth century, the prevailing theory was the blending inheritance, which was similar to the mixing of two different color paints: progeny were expected to have traits that were a blend of those of its two parents.
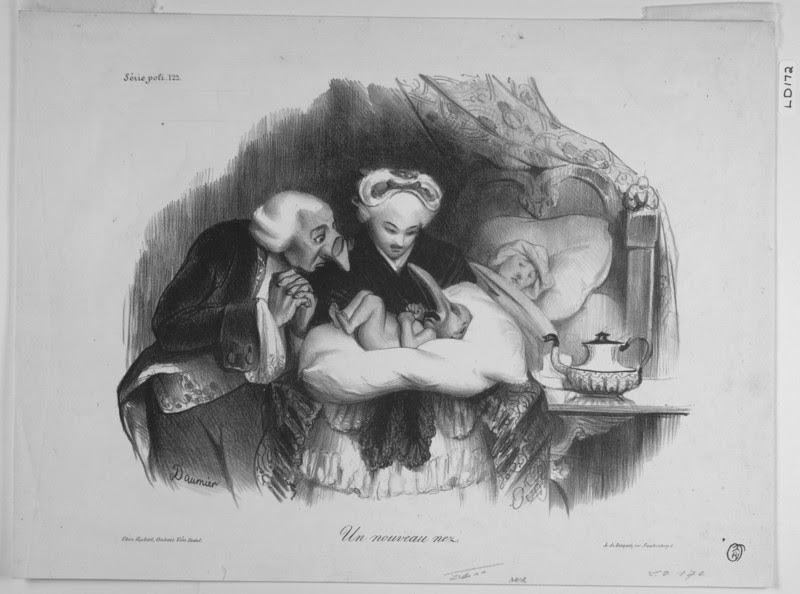
Darwin was puzzled about the mechanism of heredity and how the substance that carries parental traits into offspring could possess two seemingly contradictory qualities: its flexibility to give rise to variations and the stability required to maintain the species. He proposed the pangenesis theory to explain the mechanism of heredity. This theory suggests that an organism continually produces a specific type of small organic particle called a gemmule that accumulates in the gonads and then is transmitted to the gametes. When gametes form embryos after fertilization, their gemmules from the parents mix like the paint of two colors. Interestingly, pangenesis was in direct contradiction with Darwin’s theory of (bio)evolution. If the principles of pangenesis were correct, then the natural variations that spontaneously arise within any species would have been an exception among most individuals. Therefore, as a result of mating between an exceptional variant with “normal” individuals (and mating of its progenies with normal members for generation after generation), the variations would gradually disappear. However, Darwin’s extensive research suggested that variations in different birds of the Galápagos Islands were maintained over generations. Eventually, Darwin realized that pangenesis does not explain evolution, and these two hypotheses—(bio)evolution and pangenesis—were contradictory. But he could not solve the riddle.
The greatest challenge to pangenesis came from the German zoologist August Weismann (1834–1914). Weismann experimented with mice to see if cutting off their tails generation after generation would cause any change in their offspring. However, the progeny of those mice had normal tails. He proposed the germplasm theory, which suggests that multicellular organisms consist of two types of cells: (1) germ cells, which are present in the gonads (ovaries and testes) and contain and transmit heritable information from parents to progeny, and (2) somatic cells, which carry out ordinary bodily functions. Thus the gametes (egg cells and sperm cells) produced by the germ cells serve as carriers of heredity information, and other cells of the body do not function as agents of heredity. This hypothesis discredited the ideas of inheritance of acquired characteristics as proposed by Lamarckism and pangenesis. In this way, the distinction between the hardwired inherited traits contained within the germ cells and the soft-wired traits acquired by the somatic cells was established.
However, it remained to be known how much biological contribution the mother and father make to their offspring and what rules govern heredity.
5.1 Gregor Johann Mendel: The Mathematics of Heredity
During Darwin’s lifetime, a clergyman in a monastery in Moravia, Gregor Johann Mendel (1822–84), studied the laws of genetics by crossing pea plants. Moravia, where Mendel (figure 5.2) was born and educated, was the center of plant crossing since the eighteenth century. As discussed in the previous chapter, various pastors took on crossing and hybridization experiments for improving crops and domestic animals. They included crossing and breeding experiments in natural science curricula in the classes they taught at schools. Thus Mendel’s curiosity and his experiments aimed at understanding the laws of heredity come as no surprise.

From 1856 to 1863, Mendel grew pea plants in the five-acre garden of the monastery and conducted about 29,000 crossing experiments. The pea proved to be the ideal plant for investigating heredity. The pea is a selfing plant, but it is also easy to perform artificial pollination on it. It has a short life cycle of two and a half months and can be grown in large numbers with very few resources. Thus it is possible to study several generations of this plant within a short period. Mendel selected seven contrasting characteristics of the pea plant (see figure 5.3) in his experiment: stem length (tall or dwarf), flower color (purple or white), pod shape (inflated or constricted), pod color (green or yellow), seed shape (round or wrinkled), seed color (yellow or green), and flower position (axil or terminal). For many years, Mendel developed purebreds by self-pollination. All the offspring produced by the selfing of purebreds are the same. For example, tall plants give rise to 100 percent tall progeny, and dwarf plants produce 100 percent dwarf progeny.
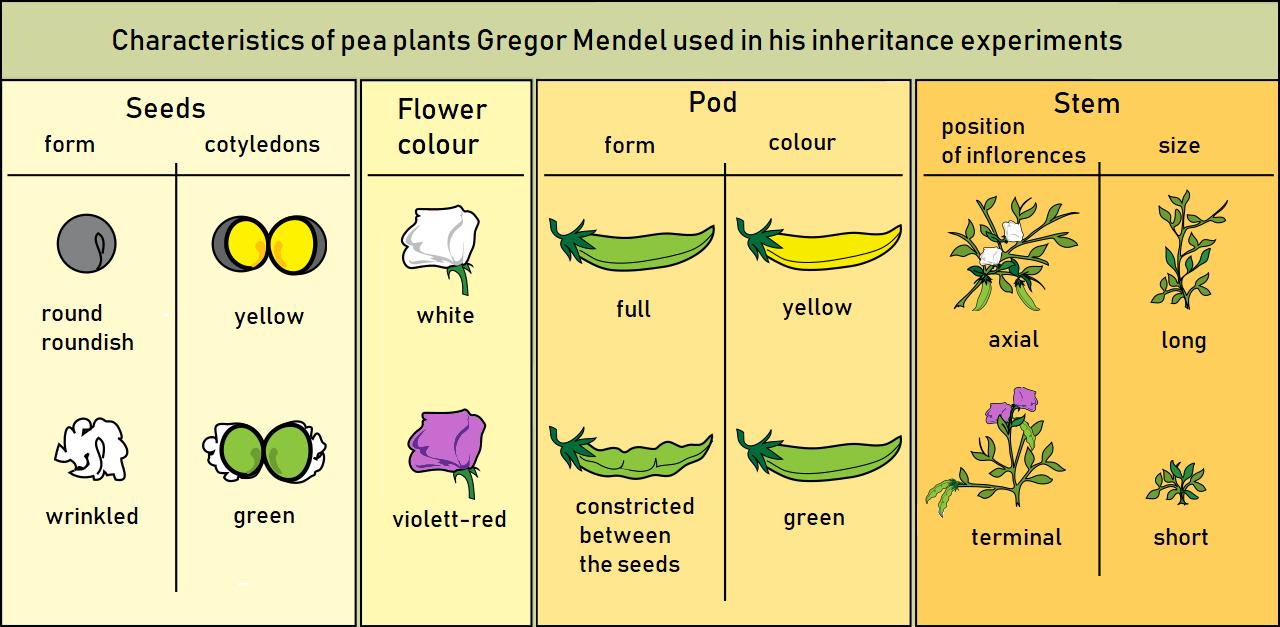
Subsequently, he used purebreds of peas for generating hybrids and for studying the pattern of inheritance of various characteristics. He observed that the crossing of the purebred purple-flowered plant with the white-flowered plant produced only purple flowers in the hybrid (first hybrid generation, or F1). He repeated these experiments on the seven pairs of pea plants that exhibited contrasting traits and found every time that all the hybrids of the F1 generation showed traits from one parent, while the contrasting trait from the other parent remained hidden. He did not observe the mixing of two contrasting traits. Based on these observations, Mendel proposed the first principle of heredity, known as the law of dominance, postulating that within any (multicellular) organism, at least two factors determine a given trait, of which only the dominant factor appears in the hybrid, and the recessive factor remains hidden or masked by the dominant trait.
In the second step, Mendel crossed F1 hybrids and analyzed their progeny (second hybrid generation, or F2) and found that 75 percent of F2 plants contained purple flowers and 25 percent of F2 plants contained white flowers (see figure 5.4). Thus after skipping the F1 generation, the white flower color reappeared in the F2 generation, but the distribution of dominant (purple) versus recessive (white) traits in their flowers was 3:1 (refer back to figure 5.1). Mendel repeated these experiments on all seven traits and always got a ratio of 3:1 between dominant and recessive traits in F2 progeny.
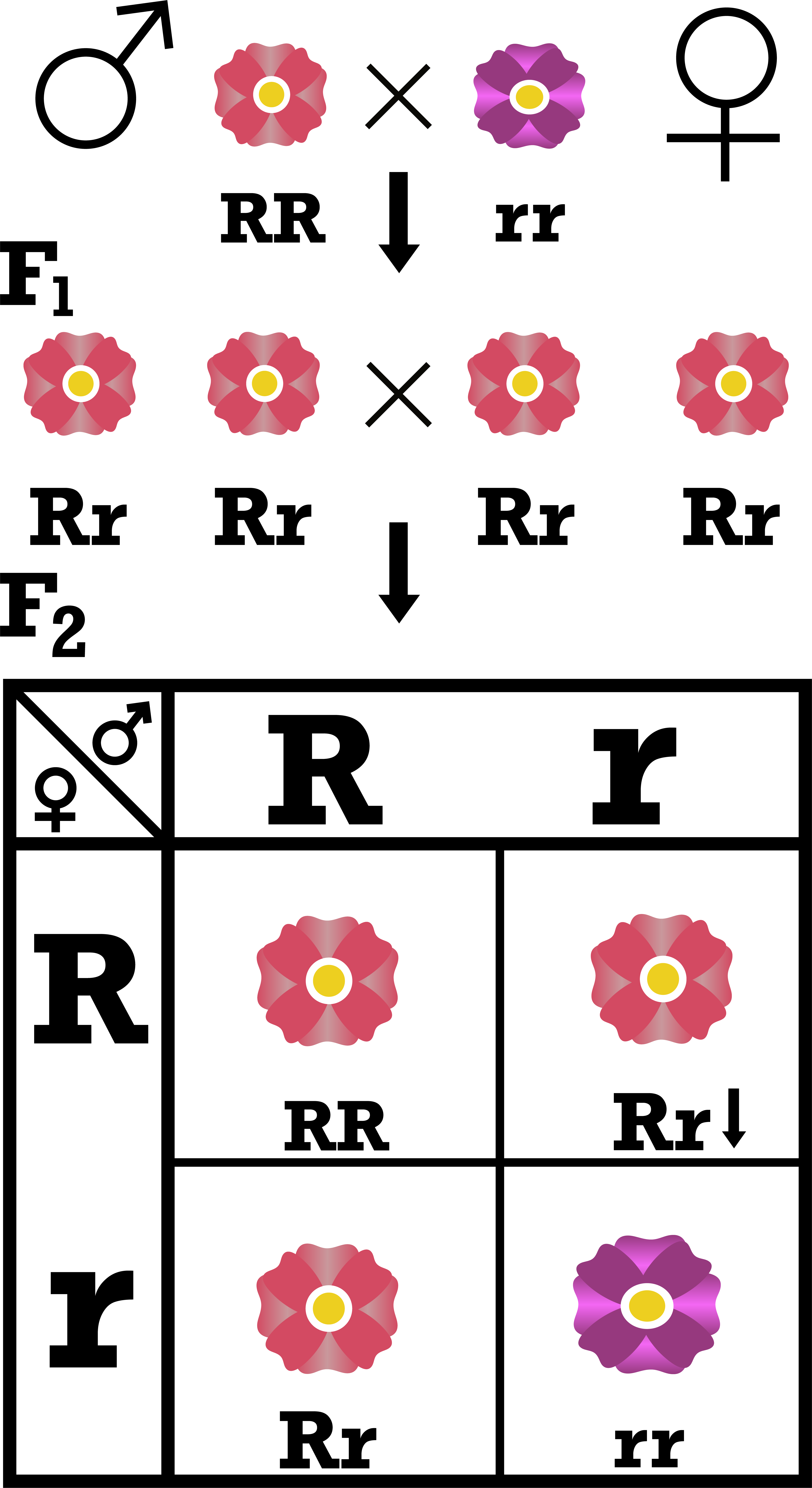
For the first time in history, it became known that the contrasting parental traits do not mix like paints of two colors; instead, they are maintained and transmitted as independent entities. During the gamete formation, the two factors separate and are distributed equally in the gametes. The fertilization between egg and sperm cells that both contain the recessive factor gives rise to the progeny containing both recessive factors, and thus the corresponding trait reappears. Mendel suggested the second law of heredity as the following:
- The individual has two copies of each factor. Each parent contributes one factor of each trait shown in the offspring.
- Some traits can mask others, but the traits don’t blend. The two members of each pair of factors segregate from each other during gamete formation.
Today, these factors are known as the alleles of a gene, which behave like alternatives to each other. Most recessive alleles cause/indicate a functional deficiency. If one allele of the pair works properly, then it hides the other’s deficiency. If both alleles of a gene are effective/functional or if at least one allele of the pair is functional, then in both cases, we see a dominant trait.
Subsequently, Mendel studied the inheritance of two different traits simultaneously. For example, he crossed homozygous plants producing yellow, smooth peas with plants producing homozygous green, wrinkled peas. As expected, the F1 generation peas were yellow and smooth; only dominant traits showed up. Mendel made crosses between the F1 individuals and found that of the sixteen plants in the F2 generation, nine produced yellow, smooth peas (resembling the dominant ancestor); one produced green, wrinkled peas (resembling the recessive ancestor); three produced smooth, green peas; and three produced yellow, wrinkled peas (see figure 5.5). In the F2 generation, he found a new combination of smooth, green and yellow, wrinkled peas in equal ratio. The overall result suggested that two different traits (such as the color and shape of a pea) are transmitted independently of each other to future generations. Mendel postulated the third law of heredity (a.k.a. the law of independent assortment), suggesting that different traits—like seed shape and seed color—are inherited independently of each other.
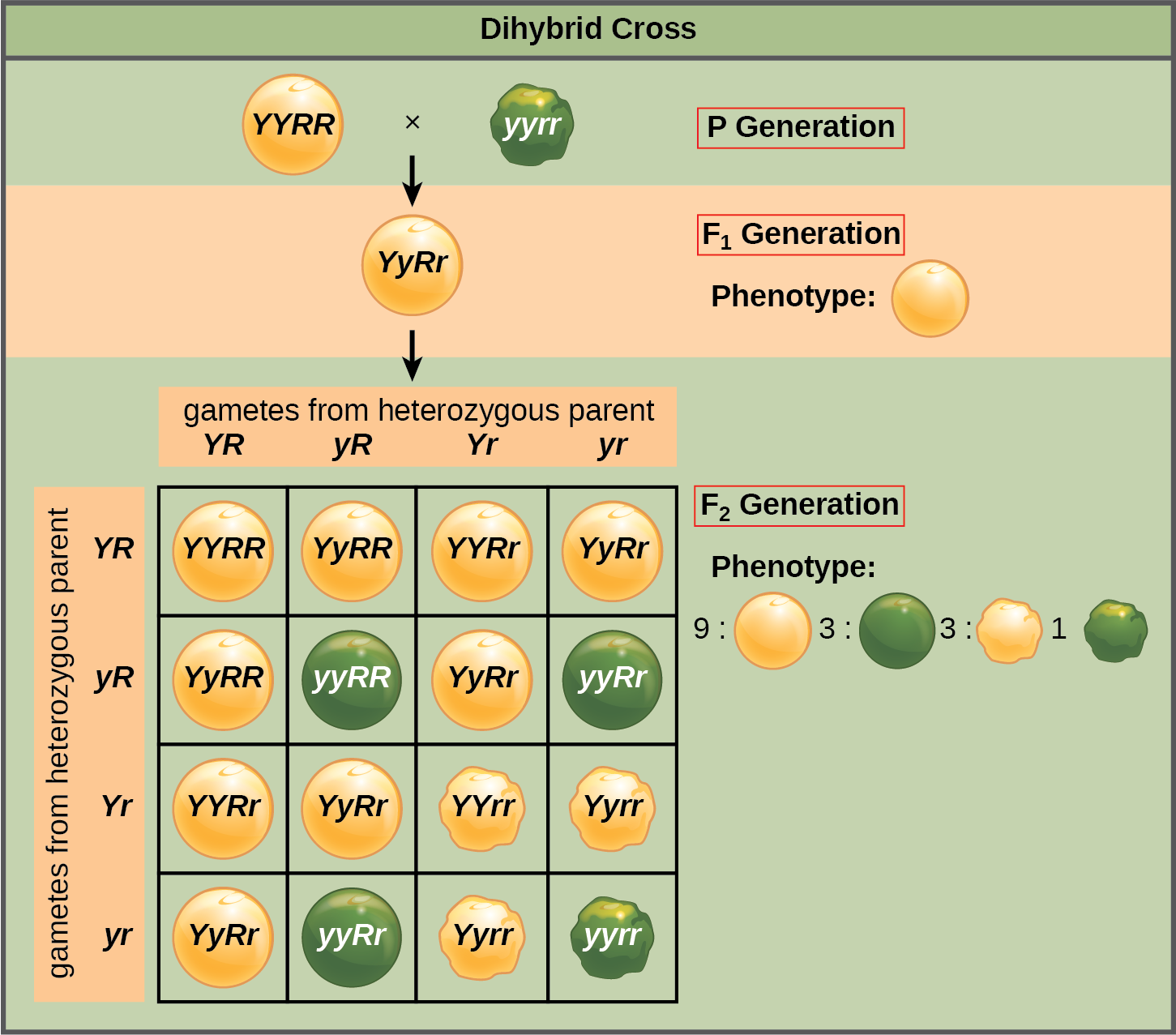
Thus Mendel’s experiment revealed the role of sexual reproduction in generating variations within the same species. Today, Mendel’s three laws are known as the fundamental principles of genetics. Mendel was not aware of the physical and chemical properties of the genetic material, but his discoveries led to the first concrete understanding of how the genetic material behaves.
Understanding the behavior of heredity required a mathematical approach rooted in deduction, the fragmentation of big questions into small workable hypotheses, and a good model system for studying heredity. Mendel, a trained meteorologist, had the ability to look at the data and apply math to it. In contrast, Darwin and most biologists of his time implemented the cataloging and description of the morphology of plants and animals. Today, Mendel is the father of genetics, but during his lifetime, his contributions were not valued by his peers. In later years, Mendel did the monastery’s administrative work, and his three principles of genetics were forgotten for thirty-five years.
Mendel’s work was no less important than Darwin’s. In fact, his work explained Darwin’s theory of evolution in concrete terms. Many people now wonder why Mendel’s discoveries were ignored, while Darwin became one of the most popular scientists during his lifetime. Perhaps, to some extent, Darwin benefited from his family background. Both his father and grandfather were well-known doctors, and he graduated from the University of Cambridge. He developed his academic network at an early age while working with John Henslow and then got an opportunity to join the HMS Beagle as a naturalist (a volunteer who was not paid a salary). In contrast, Mendel was born into a peasant family. After his initial education in the village, he became a monk and pursued further education in the monastery. During his youth, he struggled to become a high school teacher and carried out experiments with pea plants in the garden. He did not get much opportunity to discuss theories with other scientists, as he was not a part of academia; none took cognizance of his work. Also, most of his contemporary scientists were naturalists involved in cataloging and describing plant and animal diversity; they lacked mathematical understanding. Therefore, most academics and scientists ignored Mendel’s published papers. We have no way of knowing if Darwin ever knew about Mendel’s research work.
5.2 The Rediscovery of Mendel’s Laws and the Birth of Genetics
In 1900, Hugo de Vries, Carl Correns, and Erich von Tschermak independently rediscovered Mendel’s work. Thus Mendel’s three laws of heredity resurfaced after being buried after thirty-five years, but even then, these principles were not easily accepted. The first few who recognized the importance of Mendel’s laws include Cambridge University biologist William Bateson. Bateson zealously lectured on the laws of heredity in European and American institutions to popularize Mendel’s work and became known as “Mendel’s bulldog.” Bateson established the first genetics laboratory in Cambridge. Bateson proposed the term genetics for a new branch of biology rooted in the Mendelian approach and the study of inheritance and the structure function of genetic material. During this period, Vavilov had joined Bateson’s laboratory and thus became the first geneticist in the USSR. Botanist Wilhelm Johannsen proposed the word gene for the Mendelian units of heredity (factor) and called two different versions of a gene-defining trait alleles, since hybrid plants show only dominant traits and appear similar to purebreds even though their genetic configuration is different. Hence in 1909, Johannsen proposed the terms genotype for the genetic configuration and phenotype for the manifested trait—that is, its outward appearance. Hugo de Vries proposed the term mutant for organisms with rare traits (whose numbers are less than 1 percent in a population or have a defect). In this way, gradually, terminology and vocabulary for genetics increased, and slowly it was established as a subdiscipline of biology.
5.3 Exceptions and Extension of Mendelian Genetics
In the early twentieth century, many scientists repeated Mendel’s experiments and began analyzing their crossing experiments using a framework provided by Mendelian genetics. Often, the laws of heredity successfully explained their results, but less frequently, exceptions were encountered, which added new dimensions to and knowledge of heredity. Here we discuss several of such examples and how they contributed to furthering knowledge in the field.
5.3.1 Partial or Incomplete Dominance and Codominance
One exception to Mendel’s first law is partial dominance. When scientists crossed the white- and red-flower varieties of the “four o’clock,” they found pink flowers in the F1 hybrids. Thus in this case, the presence of a dominant allele did not completely mask the recessive allele, and the phenotype of the heterozygous F1 hybrid differs from its homozygous dominant parent. Furthermore, the selfed progeny of heterozygous F1 hybrid segregated in a ratio of 1 red: 1 white: 2 pink. However, the pink flower in the hybrid is not a result of the mixing of red and white but is due to the diminished quantity of red pigment. In fact, both allele coding for red and white are transmitted from one generation to another as discrete factors, but the red flowers are produced by plants where both factors are functional. When only one of the two works, only half the pigment is formed, and the pink color is seen.
Another exception is codominance, where both alleles (factors) determining a trait are functional. For example, in people with AB blood group, both A and B alleles are equally active and produce A and B antigens. A third allele, i, is also found in some people that do not produce any antigen due to mutations. Therefore, people with AA or Ai have blood group A; those with BB or Bi have blood group B, and others with ii have blood group O (no antigen).
Mendel proposed the basic rules of genetics based on the assumption that there are two alleles (dimorphic) of any gene. While it stands true for an individual, multiple alleles for the same trait exist within any population of a plant or animal. Many genes have several common alleles (they are polymorphic), which may show a complicated pattern of dominance and can be placed in a hierarchy. The interactions of these various alleles cannot be understood without concerted efforts of crossing and establishing a dominance series. For example, if we look carefully at the whole lentil grain, there are many patterns of small or big dots on the light-colored surface. If a lentil with a clear surface is crossed with one with a dotted surface, then the F1 hybrid shows a dotted trait. However, a crossing of the dotted lentil with spotted lentil plants produces an F1 hybrid showing a spotted pattern (here the dotted trait behaves recessively). Based on the results from such crossings, the dominance hierarchy of different alleles can be prepared, and then the results can be explained using Mendel’s laws. But without understanding this hierarchy, the results cannot be explained. It is noteworthy to mention that in such cases, the dominance relations affect only the correspondence between genotype and phenotype; however, the alleles still segregate and unite randomly and follow the Mendelian pattern. The difference lies in the complex relationship between genotype and phenotype.
5.3.2 Lethal Mutations
There are many essential genes found in a living organism, known as the housekeeping genes, that are required for that organism’s survival. Some of these genes are active in all cells of an organism, whereas others act specifically in a particular type of cell, tissue, or organ. Mutations in housekeeping genes can be lethal. Sometimes heterozygotes exhibit a phenotype or a disease, which leads to recessive homozygotes dying (and thus a progeny class is eliminated). In these cases, we do not find the segregation of F2 progeny according to Mendel’s laws.
In contrast, the gametes (egg or sperm cells) contain only one allele, and thus the gametes carrying a nonfunctional allele of housekeeping genes are destroyed. As a result, only the gametes carrying the functional alleles are transmitted to the next generation. In such cases, the results of the crossing do not fit into the Mendelian hypothesis, and only the functioning allele is seen in the progeny. However, close observation can reveal decreased pollination and lower seed formation. In other instances, after successful pollination and fertilization, the embryo does not develop because both alleles of a housekeeping gene required for embryonic development are mutants. Similarly, some plants die after seed germination due to a lack of functional alleles (e.g., albino mutants that lack photosynthesis capacity). There are also instances where both alleles are necessary for the normal development of an organism. For instance, in healthy individuals, both alleles of the fibroblast growth receptor gene function, but people with a mutant allele suffer from the most common form of dwarfism (known as achondroplasia, with a normal-length body but shortened limbs).
5.3.3 Pleiotropy
Sometimes a single gene affects several unrelated phenotypic traits in the same organism, and this phenomenon is known as pleiotropy. For example, specific mutations in the hemoglobin B gene cause changes in the shape of red blood cells (from round to sickle shaped) that causes sickle cell anemia (termed this to differentiate it from dietary deficiency and other causes of anemia). Due to their shape, these cells obstruct the smooth flow of blood and have a short life compared to normal cells. As a result, people carrying the mutant gene become anemic. In addition to anemia, an enlarged spleen, muscle and heart pains, a weak immune system, resistance to malaria, and early death are associated with the mutations in the hemoglobin B gene.
5.3.4 Penetrance or Expressivity in Phenotype
Researchers also noticed that certain traits are expressed fully only under a certain environment. Thus two new terms were added:
- Penetrance is whether a trait is expressed or not.
- Expressivity is the degree to which a trait is expressed (fully or partially).
We see many such examples in our day-to-day lives. For instance, genetically identical hydrangeas growing in soils of different acidity (different environments) produce flowers of a different color. Many houseplants change color if their exposure to light changes or the temperature changes. We also find different pigmentation in cats, dogs, and other animals, where the colder body parts are darker while the warm body parts are lighter.
5.3.5 Epistasis
In many instances, a single phenotype is controlled by the interaction of two or more genes. This phenomenon is known as epistasis. The epistatic genes may code for proteins involved in different steps of a biosynthetic pathway or may have an additive function. In such cases, we see alterations in F2 segregation ratios. Examples of such interactions can explain the various sizes and shapes of squashes, the different skin colors of onions, or the diversity in flower colors of many plants.
Unlike animals, plants contain many duplicate genes, and some of these have redundant and/or additive functions. Thus when both alleles of a gene are mutated and are nonfunctional, we see no phenotype due to the presence of a duplicated gene that functions properly. In such cases, a phenotype is only visible if both genes (all four alleles) become nonfunctional. In contrast, the opposite is also true: sometimes, a phenotype is only visible when two or more duplicate genes are simultaneously functional in the organism. For example, for zucchini, two genes (four alleles in total) determine the fruit size. If both genes work or at least one allele of both works, then a very small disk-shaped fruit is produced. If only one gene (or one of its alleles) is functional, then a big, rounded fruit is produced. If both genes (all four alleles) become nonfunctional, then a long fruit is produced. Here crossing between the long and flat breeds of zucchini will give rise to an F1 hybrid bearing round fruits. And the selfing of F1 progeny will result in the segregation of this trait in F2 progeny as 9 disk shaped (A_B_):6 round (A_bb or aaB_):and 1 long (aabb).
5.3.6 Genetic Linkage and Chromosome Theory
Mendel was extremely fortunate with the plant model he selected for deciphering the basic laws of heredity. He chose seven traits of pea plants that gave a consistent pattern of dominance and recessiveness between two contrasting alleles. Many of Mendel’s contemporaries could not find such a great experimental model or traits, and due to many deviations, their efforts were derailed. Even Bateson, the biggest supporter of Mendelian genetics, could not find an easy path and got entangled with unexpected results not explainable by Mendel’s laws. In 1905, Bateson and Reginald C. Punnett made a dihybrid cross between purebreds of sweet pea plants, where the dominant parent produced purple flowers and long pollen grains (PP LL), and the recessive parent produced red flowers and round pollens (pp ll). Bateson and Punnett observed that the F1 hybrid had purple flowers and long pollen grains (Pp Ll), as expected.
However, the F2 population generated by the selfing of F1 hybrids showed a skewed ratio that did not match what was expected based on Mendel’s second law of independent assortment of two independent traits. The data clearly showed that the characteristics of pollen size and flower color did not transmit independent of each other from parents to offspring. The expected ratio of the dihybrid cross was 9:3:3:1, but the results did not fit this pattern. As shown in table 5.1, the gene coding for flower color and pollen shape appears to be linked to some extent: the two phenotypic classes (purple flower + long pollen and red flower + round pollen) are larger than expected, and the number of recombinants is less than expected (1). Bateson was puzzled and could not explain these results in terms of epistasis either. However, Bateson and Punnett proposed that the F1 hybrid produced more P L and p l gametes due to some sort of physical coupling between the dominant alleles P and L and between the recessive alleles p and l (1).
Table 5.1: The results of the di-hybrid cross in sweet peas observed by Bateson and Punnett)
Phenotypes | Observed (12.3:1:1:3.4) |
Expected (9:3:3:1) |
---|---|---|
Purple flower and long pollen (dominant traits; PP LL+Pp Ll) |
4831 | 3911 |
Purple flower and, round pollens (recombinant; PP ll+pP ll) |
390 (17.8%) | 1303 |
Red flower and long pollen (recombinant; pp LL+pp Ll) |
393 (17.7%) | 1303 |
Red flower and round pollen (recessive traits; pp ll) |
1338 | 435 |
Total plants | 6952 | 6952 |
American scientist Thomas Hunt Morgan chose the fruit fly (Drosophila melanogaster) to study genetics. The fruit fly has several advantages for this study, including a short life cycle (forty to fifty days), easy propagation within milk bottles, and being amenable to the crossing. Also, with the help of a microscope, changes in the structure of the fruit fly can be easily identified. Thus it was a cheap and accessible model for the study of genetics and since then has served as an important model organism to study eukaryotic genetics. When Morgan crossed a female fly containing a purple eye (pr) and a normal wing (vg; both dominant traits: pr/pr.vg/vg) with a male fly containing a red eye (pr+) and the vestigial wing (vg+; both recessive traits: pr+/pr+.vg+/vg+), he got an F1 hybrid with the purple eye and the normal wing (pr/pr+.vg/vg+), as expected. Then he made a backcross between the F1 hybrid female (pr/pr+.vg/vg+) and the recessive male parent (pr+/pr+.vg+/vg+) (2). In these experiments, the recessive male can only produce one type of gamete, one that carries recessive red eye and vestigial wing traits. Thus the alleles contributed by the F1 female specify the F2 progeny. If both traits are sorted independently of each other, the expected ratio of these traits in the F2 progeny would be 1:1:1:1, representing both parental genotypes and two new recombinant classes.
As shown in table 5.2, he found unexpected results: a much larger number of offspring with purple eyes and normal wings or red eyes and vestigial wings was obtained in the F2 progeny. Like Bateson, he also observed a linkage between two independent traits: the linkage was not absolute, and the two types of recombinant class of progeny were in a similar proportion.
Table 5.2: The results of fruit fly backcross observed by Morgon
Genotypes and Phenotypes | Observed |
---|---|
pr+/pr. vg/vg+ (pruple eye+ normal wing) |
1195 |
pr+/pr+. vg+/vr+ : (red eye+ vestigial wing) |
1339 |
pr+/pr+. vg/vg+ (reg eye +normal wing) |
151 |
pr+/pr. vg+/vg+ (purple eye + vestigial wing) |
154 |
Total | 2839 |
The progress in the field of cytology[1] helped us understand the phenomenon of linkage between genes that were observed by Bateson and Morgan independently. From the seventeenth century onward, scientists studied the structure of different organisms through microscopes and understood that organisms are made of one or more cells. The simplest forms of life, such as bacteria, are made of only one cell (unicellular), and various animals and plants are made of many cells (multicellular). Therefore, the unit of the structure and the function of life are the cell. In the late nineteenth century, high-resolution microscopes became available, which allowed the identification of various subcellular structures. It was natural that scientists started probing the location of genetic material within the cell. In 1879, Walter Fleming noticed a fine, threadlike structure in the center of salamander cells that shrunk and assumed a clear shape during cell division that was named chromosomes. It was clear from a series of studies that the number of chromosomes is constant for a species, and chromosome numbers vary between species. Typically, multicellular organisms have two copies of each chromosome (two sets of chromosomes) within the somatic cells, and the mother and father each contribute one set of chromosomes to their offspring. For example, human somatic cells have 46 chromosomes (23 pairs; diploid). In contrast, one set of chromosomes (23 chromosomes) is present in the gametes (eggs and sperm cells).
At the time of cell division, chromosomes first double in number and then divide equally into two daughter cells. In this way, two cells are made from one mother cell, and both daughter cells have the same number of chromosomes as their mother cell. In contrast, the cell division of a mother germ cell gives rise to gametes that only contain one set of chromosomes (haploid) and thus only one copy of each chromosome. During sexual reproduction, the fusion of male and female gametes produces a diploid embryo, and the two sets of chromosomes are restored in progeny. The behavior of chromosomes during the cell division of the germ cell grossly reminds us of Mendel’s laws.
By the beginning of the twentieth century, it was recognized that chromosomes play a role in transmitting genetic traits from parents to offspring. Incidentally, the seven traits chosen by Mendel for his crossing experiments are encoded by seven genes that reside in seven different chromosomes, and thus he did not observe linkage. Therefore, Mendel did not see any contradiction in his experiments. However, besides gene linkage, Bateson and Morgan made another important observation that the linkage between two genes was not absolute and that a few recombinants are present in the F2 population. Morgan hypothesized that during germ cell division (meiosis), homozygous chromosomes interchange small regions before being divided into daughter cells, and thus the linkage between two genes located on the same chromosome occurs, resulting in the formation of recombinants (2). Thus based on the findings of cell biology and results from his laboratory, Morgan provided an explanation of the linkage between genes (2). He proposed the following:
- Genes occur in a linear order on chromosomes, and their location on chromosomes is fixed.
- Genes on the same chromosome are linked together and do not exhibit an independent assortment.
- Genes can be exchanged between chromosomes during meiosis.
- The closer genes are located on a chromosome, the less likely they will separate and recombine in meiosis.
- Chromosomes undergo segregation and independent assortment. Therefore, genes on different chromosomes follow the law of independent assortment.
5.4 Chromosome Mapping
Several experiments in Morgan’s laboratory revealed that the closer the two genes are, the stronger their association. As the distance between the two genes increases, the probability of crossing over increases, and the number of newly combined progeny (recombinant) increases in the same proportion. Thus the distance between two genes can be estimated by the number of recombinants present among the total progeny. By this method, the distance between genes can be measured in centimorgan (cM). Thus a distance of 1 cM between the two genes meant that in such cases, 1 percent of F2 progeny (of dihybrid cross) would be recombinant. Morgan’s laboratory generated a vast amount of fruit fly crossing data. Eventually, one of his students, Alfred Henry Sturtevant, analyzed this data and constructed the first genetic map of fruit flies’ chromosomes one by one. Subsequently, this method was applied to many other animals and plants to generate their chromosome maps.
Morgan did not directly observe the reciprocal exchange of segments between homologous chromosomes, but the assumptions he made about crossing over during meiosis proved to be correct. The crossing over (see figure 5.6) was experimentally confirmed almost two decades later by Barbara McClintock. But by then, scientists had constructed genetic maps of many organisms by following Morgan’s lead. Genetic maps proved to be very useful for breeders. Genes whose phenotypes are visible served as a marker for selecting other nearby/adjacent genes that had no visible phenotype but contributed to important agronomic traits. Similarly, if one desired gene is linked to another undesirable gene, with the help of a genetic map, it became possible to estimate how many crossings will be required to break this linkage.
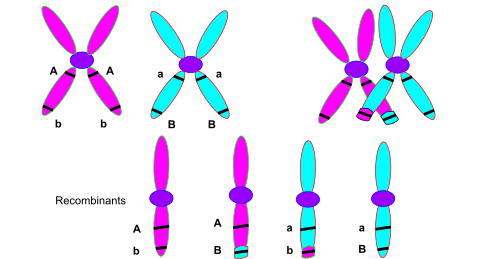
5.5 Polyploidy
The study of the cells of various animals and plants revealed that the number of chromosomes within a species is fixed. Moreover, most animals are unable to tolerate a slight change in chromosome numbers. Usually, embryos that have lost or gained one or more chromosomes are unable to survive. Unlike animals, plants have a tremendous capacity to harbor multiple sets of chromosomes; this phenomenon is known as polyploidy. Many plant species have two (diploid), three (triploid), four (tetraploid), six (hexaploid), or eight (octoploid) copies of the entire set of chromosomes. Many polyploid crops have come into existence by spontaneous hybridization events between two closely related species naturally. For example, there are 28 chromosomes within emmer wheat (Triticum dicoccoides), 14 of which are from diploid einkorn wheat (Triticum Urartu; AA genome donor) and 14 from a diploid goat grass related to Aegilops speltoides (the BB-genome donor). Therefore, emmer wheat is a tetraploid (AABB) species. Another natural hybridization event between T. dicoccoides and Aegilops tauschii (the DD-genome donor) gave rise to hexaploid modern bread wheat (Triticum aestivum; AABBDD). Thus hexaploid common bread wheat has 42 chromosomes, of which 28 are from the emmer (AABB) and 14 are from A. tauschii.
Often the increased ploidy has a direct effect on the structure of the plant in the form of an increase in the size of the leaf, fruit, or grain—thus it adds to the agronomic value of a crop. Multiplication of chromosomes in emmer and common bread wheat had resulted in an increase in grain size and greater tolerance for adverse environmental conditions. Similarly, as discussed in an earlier chapter, the octoploid ananassa strawberry (8 sets of chromosomes) is much larger than diploid wild strawberry varieties. Sometimes, breeders also create sterile hybrids by crossing two closely related species of different ploidy levels that disrupt the formation of seeds in hybrids. The seedless watermelon (a triploid) is one such example that was made by crossing a tetraploid with a diploid variety.
5.6 Summary
In later decades of the nineteenth century, a tremendous amount of geological and biological data suggested that both the earth and the living species inhabiting it have changed over time; in the process of adapting to new environments, new species emerge from the previously existing species, and some existing species also disappear. Mendel extended the knowledge by postulating three fundamental laws of heredity—which provided a correct theoretical explanation for Darwin’s theory of evolution—to describe the origin of species in the natural world. Many new discoveries in cell biology led to the birth of genetics, a subdiscipline of biology that studies heredity and the physical and structural properties of genetic material. By 1900, cells and chromosomes were sufficiently understood to give Mendel’s abstract ideas physical context. It was discovered that chromosomes contain the genes that code the various traits of an organism and that during germ cell division, the reciprocal exchange of chromosomal segments further adds to genetic diversity within a species. The observation of recombination frequencies was exploited to construct chromosomal maps and decipher the location of genes on various chromosomes and their physical relationship with one another. Chromosomal maps provided breeders with the tools for designing a rational experiment for creating improved varieties of plants and animals.
References
Bateson, W., & Saunders, E. R. (1902). Experimental studies in the physiology of heredity. In Reports to the Evolution Committee of the Royal Society. Harrison & Sons. (↵ Return 1) (↵ Return 2)
Morgan, T. H. (1909). What are “factors” in Mendelian explanations? American Breeders Association Reports, 5, 365–68. http://www.esp.org/foundations/genetics/classical/thm-09.pdf (↵ Return 1) (↵ Return 2) (↵ Return 3)
Further Readings
Landmarks in the History of Genetics. http://www.dorak.info/genetics/notes01.html
Mendel, J. G. (1865). Versuche über Pflanzenhybriden Verhandlungen des naturforschenden Vereines in Brünn, Bd. IV für das Jahr. Abhandlungen. 3–47 [for English translation, see http://www.mendelweb.org/Mendel.plain.html].
Mitosis and Meiosis Simulation (video for cell division of two types). https://www.youtube.com/watch?v=zGVBAHAsjJM
Winther, R. (2001). August Weismann on germ-plasm variation. Journal of the History of Biology, 34(3), 517–55. https://doi.org/10.1023/A:1012950826540
- The following are major discoveries in cell biology that played important role in the progress of genetics: 1839: M. J. Schleiden and T Schwann develop the cell theory. 1866: E. H. Haeckel (Häckel) hypothesizes that the nucleus of a cell transmits its hereditary information. 1869: Friedrich Miescher isolates DNA for the first time. 1879: Walter Flemming observes mitosis. 1902: W. S. Sutton and T. Boveri independently propose the chromosome theory of heredity: a full set of chromosomes are needed for normal development; individual chromosomes carry different hereditary determinants; and independent assortments of gene pairs occur during meiosis. ↵