Section 2.1: What Is Biodiversity?
We typically describe biodiversity as a thing. We marvel at places that have a lot of it, worry about losing it, and research ways of saving it. But it’s too much to ask a single word to represent the most intricate and complex aspect of our planet. Biodiversity isn’t a single thing. It encompasses all the different ways that we have thought of for describing how life varies. There are probably as many ways of describing that variation as there is variation itself. This chapter won’t go into all those intricate details, but there are a few key aspects to keep in mind.
All quantitative descriptions of biodiversity involve four interconnected components (Fig. 2.2). First, there are various metrics of variation that are the basic data units of variation. Those data are summarized using a range of statistics that describe the data in different ways. Each of the metrics is also always observed with respect to specific levels of biological organization and spatial scales.
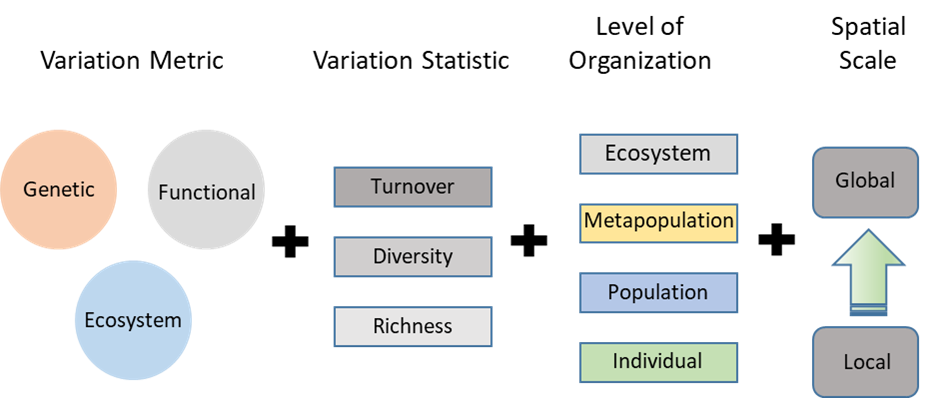
Variation Metrics
There are three broad classes of biological variation: genetic, functional, and ecosystem. Each class includes a variety of different metrics that use different base units and describe different aspects of the variation. Table 2.1 lists a few of the more common metrics.
Classes of Biodiversity Measurements | ||
---|---|---|
Genetic Metrics | Functional Metrics (i.e., behavioral/observable) | Ecosystem Metrics |
alleles species phylogenetic distance |
||
functional traits (constitutive defense, incisors, etc) life history culture |
habitats (health and connectivity) land-use types ecosystem process |
Genetic Variation
Genetic variation is the most fundamental form of biodiversity. DNA (deoxyribonucleic acid) and RNA (ribonucleic acid) sequences regulate the form and function of all life. As a result, variation in these sequences underpins much (but not all) of the other forms of variation that constitute biodiversity. Alleles (the different sequence versions of a gene) are examples of genetic variation. Up until the past decade or so, we only had the technology to describe variation in a few genes in a few organisms. But we can now sequence entire genomes relatively quickly and inexpensively, which has led to an explosion in the amount of genetic sequence variation that we have described and quantified.1
Although you may not think about them in this way, species are another metric of genetic variation. Species are genetically distinct units (more or less) whose component individuals are linked by shared heredity. Before we understood DNA or had the tools to describe its variation, we classified organisms into distinct species based on their physical traits and inferences about their degree of relatedness. One of our great scientific advances was understanding that the physical trait-based groupings reflected underlying patterns of variation in DNA and RNA sequences. Even with advances in DNA sequencing, species are still the basic file folder of life and the most tangible form of biodiversity. As of 2022 we have described and cataloged 2.2 million species,2 although the total number of species that currently exist is still just a moderately informed guess. While most estimates place the number of multicellular species in the 8-10 million range, some suggest there could be as many as 100 million eukaryotic species.3 Those estimates probably undercount the tinniest organisms, and they ignore microbial species altogether; one estimate is that there could be as many as 1 trillion of those.4
Phylogenies describe how similar (or different) genetic groupings such as species are. They also depict (or at least infer) the pattern of evolutionary relatedness among groups. Figure 2.3 is a phylogenetic tree of life based on genome sequence variation. Short branch lengths and dense nodes indicate closely related groups whose genetic sequences are more similar, while long branch lengths and distantly separated nodes indicate less genetically similar and more distantly related groups. The variability in relatedness among a collection of taxonomic units is called phylogenetic diversity.
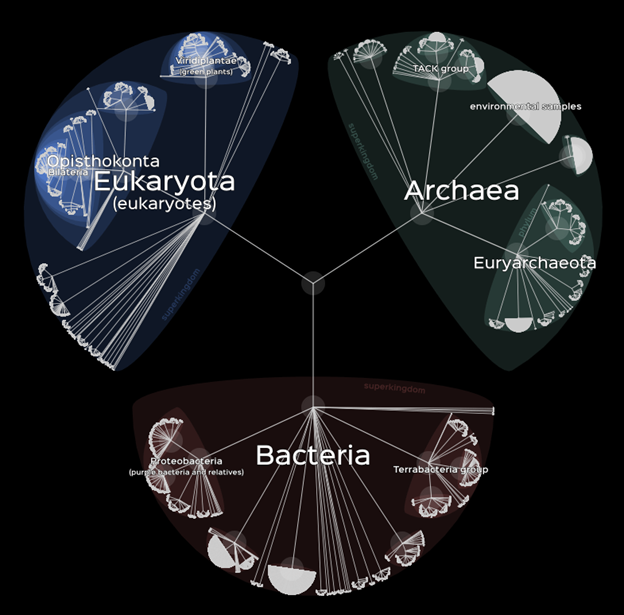
Functional Variation
Despite the fundamental role that genetic variation plays in regulating life, it is rather disappointing as a descriptor of life’s variability. Genome sequences, species lists, and phylogenies do a poor job of describing how organisms interact with the world. A species checklist is a lot less interesting than an illustrated field guide complete with descriptions of cool life history and interesting behavior. Variation in these functional attributes—functional variation—is another aspect of biodiversity.
Quantifying interactions is a bit more ambiguous than tallying differences in DNA sequences. One approach is to identify functional traits that directly relate to how organisms interact with each other or the physical environment. These can be physical traits such as color, size, or hairiness. They can be physiological processes such as growth rate or respiration. They can be behaviors such as shyness or intelligence. They can also be cultural knowledge that gets passed down from generation to generation through social learning instead of through genetic heredity.5 Examples of cultural traits include knowledge about where the best feeding grounds are, as well as more lyrical traits such as a cool new song that spreads across humpback whale populations.6
An important aspect of functional variation is that it does not directly correspond with genetic variation. For example, strong selection often causes genetically distant species to converge in traits so that they interact with the environment in similar ways. See the examples of convergent evolution discussed in Chapter 3 (see Fig. 3.7). Conversely, phenotypic plasticity can cause individuals and populations to diverge in traits even though they are genetically similar or even identical. For instance, many organisms have inducible defenses against predators, such as spines or chemical deterrents that they can switch on in the presence of predators (Fig. 2.4).
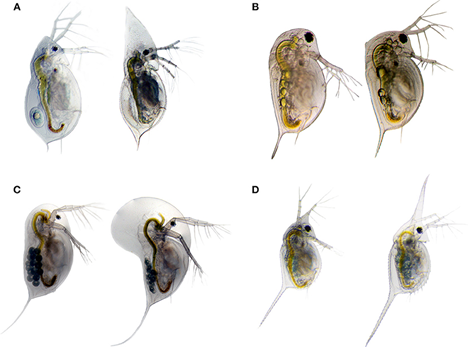
Functional variation is the most direct description of the ecological functions and processes occurring at a given place. For instance, if you were interested in understanding what factors cause primary productivity to vary across a landscape, looking at the variation in plant traits that influence photosynthesis such as leaf thickness and water use efficiency would be more informative than simply looking at the variation in plant species. Functional traits also reflect evolutionary adaptations to the various constraints and trade-offs that organisms face in trying to survive, grow, and reproduce. The constraints and trade-offs canalize functional variation into distinctive life history strategies. Examples include perennial versus annual plants, carnivores versus herbivores, and semelparity versus iteoparity. Variation in life history strategies is another metric of functional variation.
Ecosystem Variation
Metrics of functional variation such as life history traits are focused on the ways that individual organisms interact with the environment. But life is also organized into collections of individuals that interact with each other and with different physical environments, known as ecosystems (see Chap. 3). Like the neighbors on your block or in your apartment building, the composition of ecosystems is largely a product of circumstance. Members come and go as circumstances or conditions change. Unlike genetic and functional variation, ecosystems are not direct products of evolution. Still, like species, they are one of the more tangible aspects of the biophysical world. Even if a person can’t identify a single species, they can still probably distinguish a grassland from a forest from a desert. In addition, like functional traits, ecosystems are often a more direct way of describing variation in ecological processes than species are. For instance, deserts have characteristic sets of functional traits (e.g., plants with fleshy leaves, animals with super strong kidneys) as well as levels of ecosystem processes (e.g., amount of primary production, degree of environmental variability) that consistently differ from those of rain forests. The number and variability of ecosystems across landscapes are therefore additional aspects of biodiversity.
Defining ecosystem types is inherently subjective. It can also be maddeningly slippery to define what the boundaries of an ecosystem are and to decide what spatial scale is the appropriate frame of reference. An alternative approach is to instead describe variability in specific ecosystem processes or characteristics that can be more easily defined and quantified using standardized units. Examples include net primary productivity, canopy height, and structural complexity. These are similar to the functional traits of individual organisms, but these ecosystem traits emerge from the collective interactions of ecosystem components as a whole.
Variation Statistics
The variation metrics described above are basic units of variability that we can quantify into data. Like other data, we can use statistical descriptions to help organize the data and enhance our understanding of it. We have developed lots of statistics to describe biodiversity data, many of them sophisticated or complicated. They generally fall into three broad types that describe different aspects of variation. Each can be applied to almost any metric across all three categories of variation:
Richness
Richness is the total number of different types in a given area of whatever variation metric you are looking at (alleles, species, functional traits, ecosystem types, etc.).
Diversity
Diversity is synonymous with, well, biodiversity. But technically it is a narrower statistic that describes a specific aspect of variation. Just tallying the number of different types leaves out information about their relative abundance. In any sample, some types are more common than other types. Diversity takes into account both the number of types (e.g., the number of species) as well as the relative abundance of each type. It’s probably easier to see what diversity statistics are trying to describe than read about it. Figure 2.5 schematically depicts two samples that have the same species richness but different species diversity.
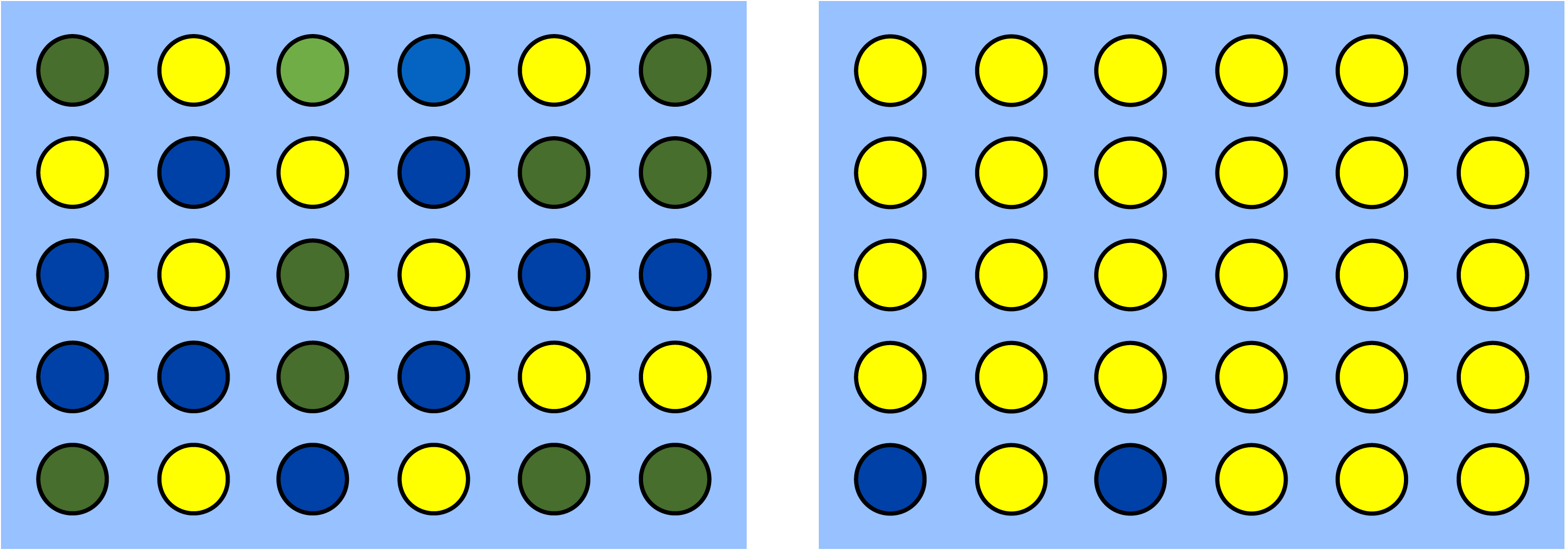
Turnover
Turnover is the degree to which variability metrics change as you move from spot to spot across a landscape. I live in an older neighborhood of single-family homes, and it seems like every home has a different landscape design and species composition. Some houses have vast expanses of manicured lawns, others are showcases for evergreen shrubs, and still others are packed full of herbaceous flower beds. Species turnover from yard to yard is high in my neighborhood. In contrast, the adjacent neighborhood is newer and composed mostly of condominiums whose landscapes are managed by a landscaping company. Those landscapes are almost identical one to another. They have the same patch of lawn, the same species of shade tree, and the same species of foundation shrubs. Species turnover from yard to yard is considerably lower in this neighborhood. Although species turnover is different, the average species richness per yard is roughly the same in each neighborhood.
Level of Biological Organization
Metrics of variation are observed and measured at distinct levels of biological organization. Most metrics can be measured at several levels, and each level provides a different perspective on the variation. DNA sequence variation is a great example. We can describe DNA sequence variation at three levels: (1) the similarity of alleles within an individual (zygosity), (2) sequence variability among individuals linked by interbreeding into local populations, and (3) sequence variability across spatially distinct populations linked by dispersal into metapopulations.
The pattern of sequence variation within and across scales reflects a dynamic balance between gene flow (dispersal + mating), selection, and genetic drift. Figure 2.6 gives a schematic example. The processes can interact in opposing or reinforcing ways, and they often have different effects at different levels of organization. For example, strong selection often reduces the level of genetic variation within local populations because any individuals that don’t have the optimal genotype get quickly selected out. At the same time, strong local selection often increases genetic variation among the populations in a metapopulation because each local population within the metapopulation evolves a unique locally adapted genotype composition. In contrast, gene flow can have the opposite influence. At the scale of the metapopulation, gene flow counteracts the effect of local selection, homogenizing genetic differences among populations. At the scale of a local population, however, gene flow from outside populations can import novel alleles, increasing levels of within population genetic variation. That probably all sounds complicated, and indeed the topic is more deserving of a population biology textbook than this one. But it is a good illustration of how the biological scale at which you observe variation can provide different perspectives. It also illustrates the dynamic complexity inherent in any description of biodiversity.
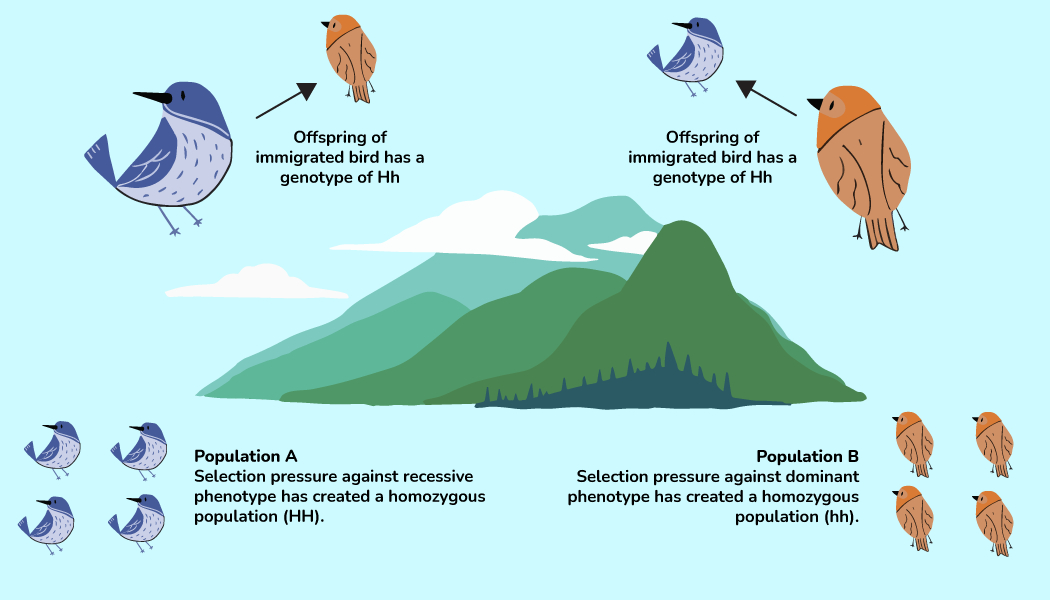
Spatial Scale
Figure 2.6 illustrates the spatial component of biodiversity. It describes variability at the local scale as well as variability across a larger landscape. To have much meaning, all descriptions of biological variation need to explicitly define what spatial scale the observation was made at. Ten species of plants sounds impressive if it is the number you have in your apartment—not so much if it is the number found across an entire continent.
In addition, how variation changes across spatial scales often reflects interesting ecological and evolutionary processes. For example, species richness increases with the size of the area in which you look for species. The positive relationship between species richness and area is one of the most ubiquitous patterns in nature.
Figure 2.7 illustrates an example of the pattern for animal species found on the Andaman and Nicobar Islands. The relationship between species and area partly reflects the effects of increasing sample size—in much the same way that a class of 100 students will have more surnames than a class of 10 students. But the shape of the relationship is also influenced by a range of ecological and evolutionary processes that are specific to different types of organisms and different landscapes. For example, species on isolated islands tend to have smaller ranges than species on more contiguous stretches of mainland. This occurs because each isolated island has evolved a unique set of endemic species with small ranges that differ from other islands, whereas species in contiguous landscapes have more room to spread out and overlap with each other.
As a consequence, species tend to accumulate with area more quickly in patchy island archipelagos than they do in contiguous stretches of mainland. Similarly, landscapes that have more different types of abiotic conditions (e.g., different soils, microclimates) or habitats (e.g., forests, grasslands, wetlands) tend to accumulate species more quickly with area and have overall higher species richness for a given area than do more uniform, less variable landscapes. Different types of organism also have characteristic shapes to their species area relationships. For instance, sedentary species like plants often have steeper species-area relationships than more mobile organism such as birds.
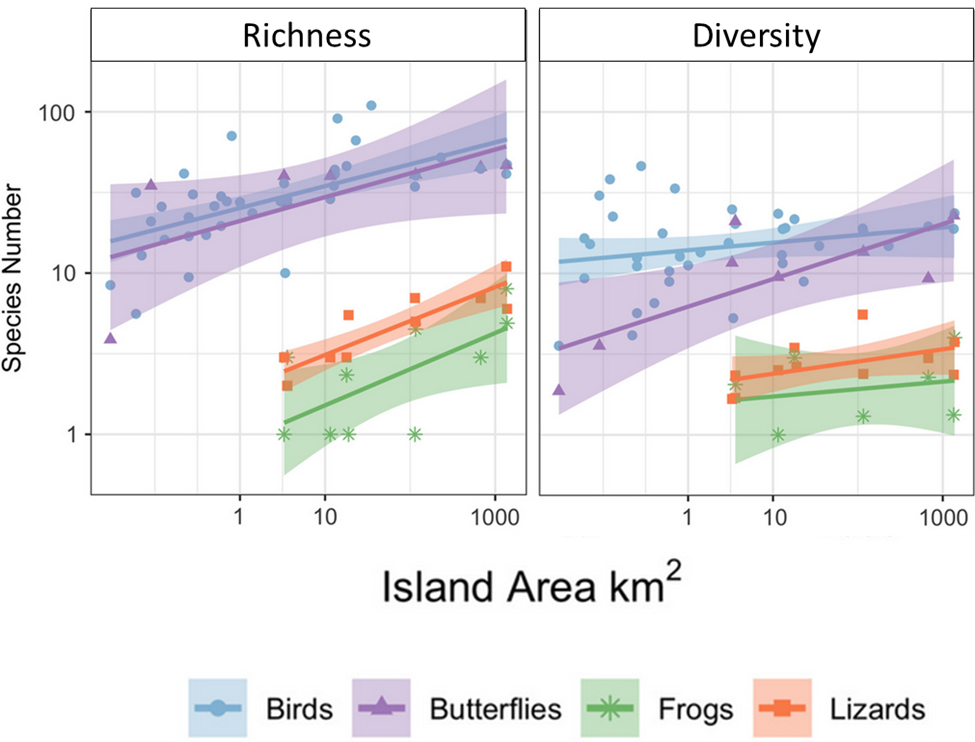